Pregnancy and postpartum transfusion
Introduction
There are many maternal adaptations and physiologic changes that occur during pregnancy. Maternal plasma volume increases to support the blood flow and blood volume necessary to sustain the placenta and fetus. Additional red cell mass is required to provide adequate maternal and fetal oxygenation. Abnormally low plasma volume expansion is associated with poor pregnancy outcomes. Hematological abnormalities are frequent in pregnancy and must be addressed to optimize maternal and fetal health. Maternal iron deficiency anemia is prevalent, and treatment is recommended to reduce the risk of postpartum transfusion. Thrombocytopenia is a common finding in pregnancy. While pregnancy-associated thrombocytopenia is often benign, serious conditions such as thrombotic microangiopathies (TMAs) and immune-mediated platelet destruction can occur and are associated with adverse outcomes. A critical review of a patient’s past medical history and careful assessment of the onset of clinical and laboratory abnormalities are necessary to establish a diagnosis and provide adequate treatment of hematological conditions of pregnancy. Physiologic changes of pregnancy pose unique challenges for women with hematologic disorders such as sickle cell disease (SCD), von Willebrand disease (VWD), and inherited bleeding disorders. Caring for women with hemoglobinopathies and bleeding disorders during pregnancy requires close collaboration between high-risk obstetric providers, hematologists, and transfusion medicine specialists.
Fetomaternal hemorrhage (FMH) can lead to maternal alloimmunization to paternally-derived fetal red cell antigens. Maternal red cell alloimmunization can affect both the sensitizing pregnancy as well as subsequent pregnancies. While the development of Rh immune globulin (RhIG) has reduced the rate of hemolytic disease of the newborn secondary to RhD alloimmunization in the middle- and high-income countries, hemolytic disease of the fetus and newborn (HDFN) due to alloimmunization to other red cell antigens such as c, E, and K also carries the risk of fetal anemia and hydrops fetalis. The blood bank plays a pivotal role in testing and guiding the management of pregnancies affected by maternal alloimmunization. Access to a safe blood supply is one of the essential components of obstetric care. Obstetric hemorrhage (OH) remains one of the leading causes of maternal morbidity and mortality globally. Comprehensive obstetric care should include early identification of women with risk factors for OH, usage of massive transfusion protocols (MTPs), and access to adjunct agents such as antifibrinolytics to prevent maternal deaths due to hemorrhage. This review explores the unique hematologic conditions, both physiologic and pathophysiologic, that occur during pregnancy and the postpartum period, summarizes management of maternal red cell alloimmunization and OH, and highlights areas related to transfusion in pregnancy and postpartum that require further investigation.
Physiologic changes in maternal blood parameters during pregnancy
Plasma volume expansion in pregnancy
Total blood volume (TBV) increases significantly throughout pregnancy. Expansion of the maternal plasma compartment drives the TBV increase during pregnancy. Plasma volume changes are detectable by weeks 6–7 of the first trimester (1). A recently published meta-analysis reported that the mean increase in plasma volume at the end of the first trimester is 6% (2). By the end of the third trimester, the average maternal plasma volume has increased by approximately 45–50% compared to pre-pregnancy baselines, equating to 1–2 L of additional intravascular fluid (2,3). Plasma volume shows the most rapid rate of expansion in the second trimester. While most studies support that plasma volume peaks in the third trimester, some suggest that maximal plasma volume is achieved in the second trimester (4,5). Women with twin and multiple gestation pregnancies have a proportional increase in the degree of plasma volume expansion (6).
Renal sodium retention and activation of the renin-angiotensin-aldosterone system primarily regulate plasma volume changes during pregnancy (7). Plasma volume increases correlate to intrauterine fetal growth and neonatal birthweight in healthy pregnancies (8,9). Low plasma volume and compromised blood volume expansion are associated with intrauterine growth restriction, maternal hypertension, and preeclampsia (PEC), as well as adverse pregnancy outcomes, including preterm delivery and recurrent pregnancy loss (10-13). Additional investigation is required to elucidate the underpinnings of the relationship between low plasma volume and these pathologic conditions of pregnancy.
As Aguree and Gernand explore (2), most studies investigating plasma volume changes in normal pregnancy were performed between 1934 and the 1980s. The majority of the study participants were of white European descent with an average maternal age in the 20s (2,3,14). Limited studies investigating how variables such as body mass index, maternal age, and parity affect plasma volume expansion during pregnancy are available. Further studies with diverse patient populations are necessary to comprehensively understand blood volume changes in pregnancy and determine whether the standard plasma volume expansion estimation of 50% applies to all women. Additionally, given the growing rate of maternal obesity and trends toward older maternal age (15,16), research developing new plasma volume reference ranges during pregnancy are required to define and distinguish physiologic versus pathophysiologic blood volume changes.
Red blood cell changes and anemia of pregnancy
Red blood cell production increases during pregnancy, with an average red cell mass reaching 20–25% above pre-pregnancy values by the third trimester (17,18). As the rate of plasma volume expansion outpaces the increase in red cell mass, physiologic anemia ensues from secondary hemodilution. The British Society for Haematology and Centers for Disease Control defines anemia of pregnancy as a hemoglobin concentration <11.0 g/dL in the first trimester and <10.5 g/dL in the second and third trimesters (19,20). The World Health Organization (WHO) outlines a uniform hemoglobin cutoff of 11.0 g/dL (21). Maternal anemia is common, affecting up to 50% of women in low and middle-income countries and between 25–50% of women in higher-income areas (22,23). The differential diagnosis for anemia in pregnancy is broad and includes nutritional deficiencies such as iron, folate, and vitamin B12, hemoglobinopathies, helminthic and malarial infections, hemolysis, and inflammatory diseases. The prevalence of different causes of anemia varies by region; however, iron deficiency anemia is the most common cause of maternal anemia worldwide (19).
To have adequate iron stores to support the enhanced erythropoiesis of pregnancy, 450–500 mg of iron is necessary. The developing fetus and placenta require an additional 350 mg of iron, and when blood losses associated with delivery are taken into account, a total of 1 gram of iron is needed to support a pregnancy (24,25). Many women have limited iron stores or are overtly iron deficient before pregnancy, leading to a reduced capacity to meet the iron requirements of pregnancy (26). Iron deficiency anemia is associated with adverse maternal and fetal outcomes. Women with iron deficiency report higher rates of fatigue and are at greater risk of postpartum depression (27). Additionally, iron deficiency anemia during pregnancy may increase the risk of postpartum hemorrhage (PPH) (28). Maternal anemia is associated with higher rates of intrauterine growth restriction, preterm birth, low APGAR scores, and increased perinatal mortality (23,29,30). Studies suggest that neonates born to women with iron deficiency anemia have neurocognitive deficits that may last into childhood, however larger longitudinal studies are required to confirm these findings (31,32).
Clinical guidelines recommend screening maternal hemoglobin levels at the initial prenatal visit and 28 weeks (19). Women experiencing symptoms of anemia or with low hemoglobin levels and red cell parameters suggestive of iron deficiency should have ferritin measurements performed. Ferritin levels <30 ng/mL are often used as the threshold to define iron deficiency; however, there are limited data to guide whether this cutoff is accurate in pregnancy (25). Elevated serum ferritin levels do not exclude iron deficiency, as ferritin is an acute-phase reactant that becomes elevated with inflammation and infection. Recent studies suggest that low serum hepcidin levels are a sensitive and specific marker for iron deficiency anemia in pregnancy and are more accurate than ferritin in the setting of systemic inflammation (33,34). Hepcidin assays are not yet widely clinically available, and additional research is needed to delineate hepcidin reference ranges in pregnancy.
Oral iron is the recommended first-line therapy for iron deficiency anemia in pregnancy. Hemoglobin should be measured 2–3 weeks after initiating oral iron replacement, and hemoglobin should increase by 1–2 g/dL after several weeks of oral iron (19,20). Suboptimal hemoglobin improvement could suggest poor oral iron absorption or an alternative etiology of the patient’s anemia, and additional investigation should be performed. Oral iron replacement should be continued until the patient’s hemoglobin is in the normal range for 3 months and through 6 weeks postpartum (19). For patients with poor oral iron tolerance, reduced enteral iron absorption, or severe anemia in the second and third trimesters, the provision of IV iron therapy is more suitable. IV iron treatment is associated with a more rapid correction of hemoglobin levels and has fewer side effects compared to oral iron supplementation (35,36). It is important to note, however, that while both oral and IV iron replacement lead to improvements in maternal red cell parameters and ferritin levels during pregnancy, limited data show that this corresponds to benefits in maternal or neonatal outcomes. A recent systematic review by Abraha and colleagues (37) determined that iron supplementation reduced maternal anemia but did not affect rates of preterm delivery, low birthweight infants, and infant mortality. As hemoglobin correction during pregnancy reduces the risks of postpartum anemia and the need for peripartum transfusion (38), the benefits of antenatal iron therapy for patients with iron deficiency anemia are currently maintained.
Thrombocytopenia in pregnancy
After anemia, thrombocytopenia, defined as a platelet count <150×109/L, is the second most common hematological abnormality in pregnancy. It has a prevalence between 6.6% and 11.6% at the time of delivery (39,40). The International Working Group (IWG) defines thrombocytopenia as a platelet count <100×109/L in pregnancy. Only 1% of the pregnant population meets the IWG laboratory criteria (41). Normal platelet counts in pregnant women range from 165×109/L–415×109/L (42). Platelet counts gradually decrease throughout pregnancy, with a platelet count nadir in the third trimester. There are several physiologic and pathologic causes of thrombocytopenia during pregnancy. When evaluating the cause of thrombocytopenia in a pregnant patient, it is essential to determine the onset of the laboratory abnormality and ascertain whether the underlying mechanism is secondary to increased platelet destruction or decreased platelet production.
Gestational thrombocytopenia (GT), also known as incidental thrombocytopenia of pregnancy, is defined as a low platelet count during the second or third trimester of pregnancy. No recent history of pre-pregnancy thrombocytopenia should be present. In GT, the platelet count usually ranges between 130×109/L–150×109/L; rarely, patients with GT may present with platelet counts below 75×109/L (41,43). Approximately 70% to 80% of pregnancy-associated thrombocytopenia is secondary to GT (41). A recent case-control study of >3,500 pregnancies found that GT was present in 12% of pregnancies (44). In GT, the mean platelet count was 134.5×109/L as compared to 208×109/L in control pregnancies. The pathogenesis of GT is unknown but likely lies on the continuum of hemolysis, elevated liver enzymes, low platelets (HELLP) syndrome, and acute fatty liver of pregnancy (AFLP) (43). Diagnosis of GT is based on clinical and laboratory parameters, as there are currently no confirmatory tests or diagnostic biomarkers for GT. In GT, thrombocytopenia spontaneously resolves after delivery, and, as such, no medical management is typically required for these patients. Importantly, GT is not associated with neonatal thrombocytopenia.
Immune thrombocytopenia (ITP) is the most common cause of platelet counts below 50×109/L during pregnancy (41,43). Approximately 3% of pregnancy-associated thrombocytopenia is caused by ITP (41,43). No diagnostic testing is available to differentiate ITP from other causes of thrombocytopenia in pregnancy such as GT; however, the majority of women with ITP in pregnancy have a history of previously diagnosed ITP (45). Maternal ITP can lead to neonatal thrombocytopenia, and neonatal platelet counts should be monitored after delivery (45).
Other uncommon causes of thrombocytopenia during pregnancy include TMAs such as PEC, HELLP syndrome, AFLP, thrombotic thrombocytopenic purpura (TTP), and atypical hemolytic uremic syndrome (aHUS) (41,43). PEC/HELLP syndrome is the most common cause of TMA-associated thrombocytopenia in pregnancy. In PEC/HELLP, new-onset hypertension (systolic blood pressure ≥160 mmHg or diastolic blood pressure ≥100 mmHg) after 20 weeks is present in addition to features such thrombocytopenia, impaired liver function or injury, new-onset renal insufficiency, pulmonary edema, and cerebral or visual disturbances (43). Delivery is the treatment of choice for PEC/HELLP, and serial monitoring of platelet counts can help guide the timing of delivery in a pregnancy affected by PEC/HELLP. If clinical and laboratory parameter improvement does not occur within 72 hours post-delivery, other TMAs should be considered. AFLP is another pregnancy-specific TMA that often occurs in a multiple gestation pregnancy. Abdominal pain, nausea, vomiting, and reduced plasma antithrombin III levels are common clinical symptoms and laboratory findings associated with AFLP. AFLP is managed by supportive care, including fresh frozen plasma (FFP) and red cell transfusions as indicated. Similar to PEC/HELLP syndrome, delivery is the treatment of choice in AFLP.
TTP and aHUS are TMAs that are not specific to pregnancy; however, pregnancy can trigger the development of both TTP and aHUS. ADAMTS13 is the test of choice to differentiate between TTP and aHUS. An ADAMTS13 activity of <10% is diagnostic for TTP. Patients with aHUS typically have elevated serum creatinine (>1.7 mg/dL), moderately reduced platelet counts (~45×109/L), and ADAMTS13 activity levels >10% (41,43). Therapeutic plasma exchange is the treatment of choice in patients with TTP, while complement inhibitor therapy is the primary treatment for aHUS.
Platelets can be prophylactically transfused when a patient’s platelet count is below 30×109/L or when bleeding is anticipated during procedures or delivery (43). Judicious platelet transfusions can be provided to patients with TTP or aHUS if clinically indicated; however, there has been historic concern that platelet transfusions could increase the risk of thrombosis in patients with TTP (46). For neuraxial anesthesia, platelet counts above 70×109/L–80×109/L are considered safe and are associated with a very low risk of complications such as epidural hematoma (42). In unstable pregnant patients, weekly platelet counts should be performed starting at 32–34 weeks of gestation (43). In high-risk patients with thrombocytopenia and bleeding, antifibrinolytics are important adjunctive therapies (43).
Maternal red cell alloimmunization and HDFN
Pathophysiology of HDFN
HDFN arises when maternal antibodies recognize paternally-derived red cell antigens on the surface of fetal and neonatal erythroid cells. HDFN represents a spectrum of disease ranging from a positive direct antiglobulin test at birth in an asymptomatic neonate with otherwise normal blood counts to severe fetal anemia and death. Transplacental transfer of maternal IgG antibodies begins within the first several weeks of life (47). Circulating maternal red cell antibodies that cross the placenta and bind fetal erythroid precursors and red cells lead to immune-mediated clearance and destruction. Subsequent fetal anemia drives increased red blood cell production through extramedullary hematopoiesis in the spleen and liver. Severe anemia leads to hypoxia and multiorgan failure, including high output heart failure. Progressive liver failure results in reduced albumin levels and decreased oncotic pressure, which, in conjunction with cardiovascular compromise, contributes to the widespread edema and ascites characteristic of hydrops fetalis. The mortality rate of hydrops fetalis remains high (48).
Red blood cell destruction leads to increased levels of bilirubin. In utero, excess bilirubin crosses the placenta and is eliminated by the maternal liver. After birth, the neonate’s liver becomes responsible for bilirubin clearance. The hepatic system for bilirubin elimination is immature at birth due to reduced amounts and activity of the enzyme uridine diphosphate glucuronosyltransferase (49). As such, neonates are at high risk of hyperbilirubinemia, particularly when hemolysis causes supraphysiologic levels of bilirubin. This risk is exacerbated in premature neonates (50). Severe hyperbilirubinemia can lead to encephalopathy known as kernicterus, which can cause permanent neurologic damage characterized by sensorineural hearing loss, cerebral palsy, and gaze paralysis if untreated (51).
Following the implementation of routine RhIG prophylaxis in RhD negative women in middle and high-income countries, ABO HDFN has become the most common cause of HDFN (52). While ABO incompatibility is present in 20–25% of pregnancies, ABO HDFN has an incidence of 1 in 150 births (53,54). In contrast to HDFN secondary to RhD incompatibility, ABO HDFN is usually mild, tends to affect the neonate rather than the fetus, and is typified by early jaundice. Naturally occurring anti-A and anti-B isohemagglutinins are IgM antibodies that are unable to cross the placenta. Group O persons can make IgG anti-A and anti-B, which form the pathophysiologic basis of ABO HDFN. IgG anti-A and anti-B are less common in persons with group A, B, or AB blood groups. Fetal and neonatal red cells express one-third the amount of A and B antigens compared to adult red cells (55,56). Additionally, A and B substances are found in the serum and on many other tissues throughout the body (55,57). Tissue and serum A and B substances compete with A and B antigens on the red cell surface for maternal antibody binding, thereby providing protection against antibody-mediated red cell destruction. These protective mechanisms can be overwhelmed in neonates with increased red cell A and B antigen expression or whose mothers have high anti-A or anti-B titers (58).
Maternal alloantibodies to more than 50 different non-ABO blood group antigens have been identified as causative in HDFN (59,60). Before the development of RhIG and the advent of intrauterine transfusion (IUT), HDFN affected nearly 1% of all pregnancies and carried a 50% mortality rate (61). RhD continues to be a primary cause of HDFN, particularly in regions in which universal RhIG prophylaxis is not available (62,63). Other Rh system antigens, such as c and E, are commonly implicated in non-ABO HDFN (64). As Kell system antigens are expressed on early erythroid precursors, anti-K1 mediated HDFN can include erythropoietic suppression and reticulocytopenia. Alloantibodies to antigens in the Duffy, Kidd, MNS, and P blood group systems have all been reported in moderate to severe cases of HDFN (60). The IgG subtypes IgG1 and IgG3 are associated with more severe HDFN (65,66). There is also growing evidence that the type and extent of IgG glycosylation affects red cell antibody pathogenicity. Kapur and colleagues (67) demonstrated that low anti-D fucosylation correlated with increased red cell phagocytosis and lower fetal hemoglobin levels. Interestingly, glycosylation patterns vary based on the antigen specificity of the antibody (68), and additional investigation is required to evaluate the effects of glycosylation patterns on non-RhD HDFN. Neither IgG subtyping nor glycosylation pattern assessments are in routine clinical use.
Maternal alloimmunization
Sensitization to red cell antigens occurs through transfusion, pregnancy, and transplant. Small volume, spontaneous FMH is common in pregnancy, and the rate of FMH increases with gestational age. Nearly half of women have detectable fetal red cells in the maternal circulation by the third trimester (64). Risk factors for FMH include invasive procedures (amniocentesis, chorionic villus sampling), external cephalic version, placental abnormalities, abdominal trauma, abortion, fetal death, and manual removal of the placenta (69). A recent multinational retrospective study aimed at assessing the impact of extended red cell antigen matching on the formation of alloantibodies found that the causative antibodies in 83% of women with a history of severe HDFN were formed secondary to previous pregnancy rather than transfusion (70). It follows that increasing parity is a risk factor for maternal alloimmunization and HDFN (71).
Levine was the first to observe that ABO incompatibility between mother and fetus reduced the rate of RhD alloimmunization in RhD negative mothers (72). The mechanism underlying the protective effect of ABO incompatibility is multifactorial and partly mediated by the enhanced clearance of circulating fetal red cells by maternal isohemagglutinins (73). Recently, Zwiers and colleagues confirmed that ABO incompatibility also protects against sensitization to non-Rh red cell antigens (74). Interestingly, the authors found that RhIG administration also correlated to reduced alloimmunization rates to non-Rh antigens, suggesting that RhIG may have an immunosuppressive effect beyond RhD. Additional maternal risk factors for red cell alloimmunization include previous transfusion, history of major surgery, including cesarean section, hematologic disease, and previous pregnancy with a male child (75).
The prevalence of red cell alloimmunization in pregnant women and women of childbearing age varies significantly depending upon the study period, geographic region, and availability of RhIG prophylaxis. Recent studies of pregnant women in Israel, England, and India showed red cell alloimmunization rates of 1.0%, 0.4%, 2.27%, respectively (76-78). The risk of red cell alloimmunization differs based on the diversity of red cell antigens within a population. For example, as the prevalence of the RhD antigen in populations of Asian ancestry is >99%, the risk of RhD alloimmunization is low (60). In contrast, approximately 15% of persons of white European descent are RhD negative, and there is a comparatively higher risk of RhD incompatible pregnancies.
The American College of Obstetrics and Gynecology (ACOG) recommends all women have ABO and RhD typing and an antibody screen to detect IgG antibodies at the first prenatal visit (79). AABB and ACOG guidelines submit that the antibody screen should be repeated at 28 weeks in RhD negative women before RhIG administration (64). Several European guidelines recommend repeat antibody testing at 28 weeks in all women regardless of RhD status (71,80). First-trimester screening alone may miss late alloimmunization. However, whether additional screening reduces adverse maternofetal outcomes requires further investigation. After obtaining a positive maternal antibody screen, it is necessary to determine whether an identified antibody is clinically significant. IgM antibodies that cannot cross the placenta, such as anti-N, or antibodies recognizing antigens that are poorly developed on fetal red cells, such as I and Lewis system antigens, pose little risk of HDFN.
Management of alloimmunization in pregnancy
Once a clinically significant maternal red cell alloantibody is identified, fetal risk for HDFN should be assessed. If biologic paternity is assured, a paternal specimen should be obtained for red cell antigen assessment. For non-RhD antigens, routine red cell phenotyping methods can be used to determine whether the father is homozygous or heterozygous for a red cell antigen and, consequently, if the fetus has a 100% or 50% probability, respectively, of carrying an implicated antigen. If maternal anti-D is present and paternal red cells are phenotypically RhD positive, genetic testing for paternal RHD zygosity is recommended to establish the fetus’s likelihood of carrying the RhD antigen. If paternity cannot be confirmed or if the father is heterozygous for the implicated red cell antigen, fetal DNA can be assessed. Fetal DNA is acquired via amniocentesis or noninvasively using cell-free fetal DNA (cffDNA) techniques. Amniocentesis is routinely available but carries a risk of adverse effects such as pregnancy loss and hemolysis (81). Circulating cffDNA is detectable by the 5th week of gestation, and cffDNA assays for fetal blood group antigen analysis have been validated from as early as 9 weeks gestational age (82). Many countries in Europe have implemented routine cffDNA methods to screen for fetal RHD (82-84). cffDNA assays for other red cell antigens including c, E, and K have also been developed (85). In the United States, cffDNA assays for fetal RHD genotyping are available through commercial reference lab testing, but there is limited availability of cffDNA testing for other red cell antigens (82). While cffDNA platforms eliminate the risks associated with amniocentesis, whether this testing reduces fetal morbidity and mortality compared to traditional serologic testing is a matter of debate.
Monitoring serial maternal antibody titers during pregnancy can provide insight into whether fetal red cell antigens are providing an ongoing immune stimulus and are often used as part of the risk assessment for HDFN. The AABB recommends performing manual tube titers in saline with antihuman globulin using a 60-minute 37 ℃ incubation (64). As the reproducibility of titers between laboratories varies widely, titers should be performed by the same laboratory throughout a patient’s pregnancy, preferably running previous maternal samples simultaneously for comparison (64,79). Using potentiators or other testing platforms such as gel is not currently recommended, as studies comparing titer methodologies support that these may result in higher titers than conventional tube testing (86). Titers are typically performed every 4 weeks if the titer is 1:8 or lower. Most centers consider a critical anti-D titer to be 1:8 to 1:32 or a 2-fold increase in titer level (79). Serial titers are most predictive of disease severity in the first affected pregnancy and are less informative in women with a previous history of HDFN (73). Additionally, critical titers for anti-K and other red cell antigens are less well defined. However, anti-K titers of 1:8 are often cited as critical given the association between anti-K and severe fetal anemia. Some institutions incorporate monocyte monolayer assays or antibody-dependent cellular cytotoxicity (ADCC) tests when assessing the immunogenicity of maternal antibodies. A recent publication by Koelewijn and colleagues showed that ADCC assay results more accurately predicted need for intrauterine or neonatal transfusion or phototherapy than antibody titers (87). In contrast, Slootweg et al. found that ADCC was not as informative as antibody titers at predicting the need for intrauterine or postnatal transfusion therapy in pregnancies affected by anti-K alloimmunization (88).
Before the last two decades, monitoring fetal anemia required invasive sampling to evaluate amniotic fluid bilirubin levels (89). The current standard of care for assessment of fetal anemia utilizes noninvasive Doppler ultrasound to measure fetal middle cerebral artery (MCA) peak systolic velocity (90). An increased peak systolic MCA velocity >1.5 times the median for gestational age predicts moderate to severe fetal anemia. When moderate to severe fetal anemia or evidence of hydrops is detected, fetal blood sampling and IUT are recommended (Figure 1) (79). Red cells for IUT should be group O, negative for the causative red cell antigen, and crossmatch compatible with maternal serum. Additionally, the red cell unit should be leukoreduced, irradiated, and hemoglobin S negative. Some standards recommend selecting fresh red cells to maximize red cell lifespan (64). However the age of red cells for IUT has not been widely studied. Accessing the umbilical vein through intrahepatic puncture or puncture of the placental cord insertion is considered safest (91). Intraperitoneal puncture can be performed if gestational age precludes access to the umbilical vein. IUT can be repeated to treat fetal anemia until the fetus reaches an appropriate gestational age for delivery.
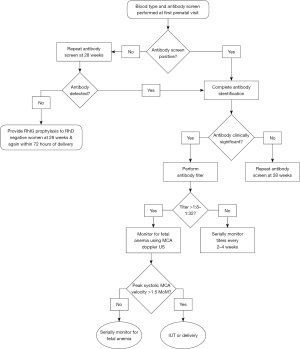
IUT performed earlier than 20–22 weeks carries a significant risk of complication and fetal death (92). Intravenous immunoglobulin (IVIg) has been used to prevent or delay the onset of fetal anemia in women with high titer alloantibodies or a previous history of HDFN. A recent multicenter, retrospective study showed that early IVIg administration delayed the onset of fetal anemia and reduced the rates of fetal hydrops and neonatal exchange transfusion after delivery in a cohort of women with a history of severe HDFN (93). Other studies support that maternal IVIg administration reduces fetal mortality in HDFN (94,95). Early initiation of maternal IVIg therapy is associated with better outcomes. Expert consensus guidelines suggest initiating IVIg by 12–16 weeks gestational age in pregnancies at high risk of HDFN (91). Therapeutic plasmapheresis (TPE) has been used as an adjunct therapy in HDFN. TPE alone showed little benefit in HDFN, likely due to antibody rebound in the absence of concomitant immunomodulatory therapy (96). TPE in conjunction with IVIg has been reported to reduce the risk of fetal anemia and prevent or delay the requirement for IUT in case reports and small case series of patients with a previous history of HDFN (97-99). Given the paucity of data on TPE in HDFN, the role of TPE in the management of HDFN remains to be determined. Maternal plasma volume calculations for TPE or IVIg administration should be adjusted for increasing plasma volume throughout gestation.
Novel treatments targeting transplacental transfer of maternal IgG are being explored in HDFN. The neonatal Fc receptor (FcRn) transports maternal IgG across the placenta and aids in IgG recycling, which increases the half-life of IgG (100). Murine models have shown that FcRn blockade reduces the transplacental transfer of IgG and protects fetuses from thrombocytopenia in a model of neonatal alloimmune thrombocytopenia (101). M281 is a recombinant monoclonal antibody that blocks the binding of IgG to FcRn (102). Human studies have demonstrated that M281 reduces serum levels of IgG without a concurrent increase in infection (103). The UNITY trial (NCT03755128) is an ongoing multicenter clinical trial investigating whether M281 modulates the requirement for IUT in women at high risk of early-onset HDFN. It is hoped that such therapeutic approaches will reduce the morbidity and mortality of HDFN when fetal anemia occurs before IUT is feasible.
After delivery of a neonate affected by HDFN, bilirubin and hemoglobin are serially monitored. Phototherapy is used to treat hyperbilirubinemia and induces isomerization of bilirubin into soluble forms that can be excreted in the urine (104). Phototherapy initiation is guided by nomograms that take into account gestational age and bilirubin levels (105). Intensive phototherapy has significantly reduced the rates of neonatal exchange transfusions (106). Exchange transfusions remove red cells coated with maternal antibodies, reduce serum bilirubin levels, and alleviate fetal anemia. Exchange transfusion is indicated if there is evidence of bilirubin encephalopathy or if bilirubin levels remain in high-risk zones despite intensive phototherapy. In double volume exchange transfusions, up to 90% of the neonatal blood volume is replaced with reconstituted whole blood (64). Adverse outcomes of exchange transfusion include thrombocytopenia, infection, and hemodynamic instability. The risk of morbidity and mortality secondary to exchange transfusion increases in preterm neonates (107).
Whether neonatal IVIg administration modulates the requirement for exchange transfusion in neonates affected by alloimmune HDFN is debated. A recent Cochrane review showed that neonates treated with IVIg for alloimmune HDFN underwent lower rates of exchange transfusion; however, the overall quality of evidence was judged to be low (108). Randomized controlled trials with a low risk of bias have not upheld these findings (109). There is also growing concern that neonatal administration of IVIg may increase risk of necrotizing enterocolitis (110). Additional studies are required to assess the safety and efficacy of postnatal IVIg for the treatment of HDFN.
Late anemia is common in neonates affected by HDFN. The etiologies of late anemia include ongoing immune-mediated destruction of neonatal red cells by persistent circulating maternal antibodies and bone marrow suppression after IUT or exchange transfusion. The majority of infants affected by HDFN require at least one top-up transfusion (111). Neonates treated with IUT have higher rates of postnatal top-up transfusions, with studies reporting that greater than 75% of neonates require top-up transfusions after IUT (111-113). The need for top-up transfusions typically falls within the first 3 months after delivery.
There have been several recent case reports suggesting that maternal immunoglobulin transmission through breast milk could contribute to prolonged postnatal courses of HDFN. In 2017, DeMoss and colleagues described a case of HDFN secondary to anti-K in which anti-K was detectable in maternal breastmilk (114). Li and Blaustein reported a case of prolonged HDFN purported to be due to passive transmission of maternal anti-D in breastmilk (115). A subsequent case series demonstrated that maternal red cell alloantibodies were present in the mothers’ breastmilk in three cases of prolonged neonatal HDFN (116). These reports merit larger, more rigorous studies to assess rates of detectable red cell alloantibodies in maternal breastmilk and to determine whether antibodies conveyed through breastmilk can bind neonatal red cells. Additional investigation is required to better understand the rates of gastrointestinal adsorption of maternal IgG, the contribution of breastmilk versus transplacental transmission of maternal immunoglobulin, and to exclude other factors that could contribute to prolonged neonatal anemia. While these case reports are intriguing, given the limited published data, no conclusions regarding whether breastfeeding is a risk factor for protracted anemia in HDFN can be made.
Prevention of maternal alloimmunization
The development of RhIG immunoprophylaxis in RhD negative women to prevent the formation of anti-D antibodies remains one of the most successful advancements in managing HDFN. RhIG is a polyclonal anti-D product manufactured from plasma obtained from pooled blood donors who have been sensitized to the RhD antigen. Recombinant forms of RhIG have been synthesized but are not yet in clinical use, as they have demonstrated variability in response and adverse effects, including hemolysis (117,118). ACOG recommends providing RhIG at 28 weeks, within 72 hours of delivery, and after occurrences that increase the risk of FMH, such as amniocentesis, pregnancy loss, and abdominal trauma (79). RhIG was initially used only for postpartum prophylaxis in RhD negative women; however, it was observed that approximately 2% of women developed anti-D before delivery (119). An antenatal dose of RhIG at 28–30 weeks is now considered standard of care, as greater than 90% of women who make anti-D secondary to fetal antigen exposure become sensitized after 28 weeks (79). A recent meta-analysis suggested that prenatal RhIG given at 28 and 34 weeks is most effective at preventing RhD alloimmunization; however additional studies that include financial analyses are required to confirm the feasibility and applicability of these findings (120). Current RhIG regimens have reduced the rate of pregnancy-related RhD alloimmunization from 16% to less than 0.1% (121).
RhIG should be provided to RhD negative women who have not been previously sensitized to RhD. If fetal blood typing via cffDNA testing confirms that the fetus is RHD negative or if the neonate is found to be RhD negative at birth, RhIG is not mandatory. Some regions now routinely use fetal RHD genotyping to guide RhIG prophylaxis in RhD negative women selectively; however, this practice is not in widespread use in the United States (122). Studies have corroborated that women with weak D types 1, 2, and 3 are not at risk of RhD alloimmunization and, as such, do not require RhIG prophylaxis (123). Cost-benefit assessments have indicated that performing RHD genotyping on pregnant women with weak D phenotypes prevents unnecessary RhIG administration and is cost-effective. Many professional guidelines recommend this be implemented as routine practice (79,124,125).
When an RhD negative mother delivers an RhD positive neonate, a maternal blood sample is required to estimate the amount of FMH and calculate the appropriate dose of RhIG. The rosette screen incubates the maternal specimen with reagent anti-D serum and group O, RhD positive indicator cells. The RhD positive indicator red cells form rosettes around RhD positive fetal cells and yield detectable positive results when 10 mL or more fetal cells are present in the maternal circulation (126). If the rosette screen is negative, a standard dose of 300 µg RhIG is given (64). If the rosette screen is positive, additional testing is necessary to quantify the FMH. The rosette test may yield a false positive in women with positive DAT or weak D variants.
The Kleihauer-Betke (KB) acid elution test relies on the principle that adult hemoglobin is sensitive to acid treatment while fetal hemoglobin is resistant. Thin smears of maternal blood are made and treated with acid, and the number of dark pink fetal cells are counted relative to the number of pale maternal cells. This ratio is then used to calculate the volume of FMH using a standard estimated maternal blood volume of 5,000 mL. One 300 µg vial of RhIG provides sufficient coverage to prevent alloimmunization for FMH volumes up to 15 mL of red cells or 30 mL of whole blood. The number of vials to be used is adjusted based on the calculated volume of FMH. Given that the KB test requires manual counting and can be influenced by variables such as thickness of the smear, there is inherent inaccuracy. As such, AABB recommends adding one additional vial to RhIG to all dose calculations (64). The KB test may overestimate the volume of FMH in women with elevated fetal hemoglobin levels due to hemoglobinopathies or hereditary persistence of fetal hemoglobin. More precise flow cytometric techniques measuring fetal hemoglobin and the RhD antigen have been developed to overcome the limitations of the KB test; however, these have yet to be implemented in many clinical practice (127).
The standard estimate of 5,000 mL may underestimate the TBV in obese women, which increases the risk of inadequate RhIG dosing. As the rate of obesity grows worldwide, studies are needed to develop better tools for estimating blood volumes and mitigating the risk of underdosing of RhIG. Guidelines now recommend using maternal height and weight to calculate TBV for FMH quantification and RhIG calculations (128). Intravenous administration of RhIG should be considered in obese women because intramuscular adsorption may be reduced in persons with significant adiposity (129). An additional population trend that raises concern is the growing number of women who decline RhIG immunoprophylaxis due to general vaccine hesitancy (52). Whether rates of RhD HDFN will increase secondary to underdosing of RhIG in obese women and RhIG refusal remains to be determined.
There are currently limited tools to prevent alloimmunization secondary to fetal antigens in pregnancy outside of RhIG. Most alloimmunization prevention strategies in women of childbearing age focus on reducing the risk of sensitization in the setting of red cell transfusion. If a woman’s blood type is not known in the setting of trauma or life-threatening hemorrhage, it is recommended to transfuse group O, RhD negative red cells until a blood type is obtained. For transfusions when a patient’s red cell phenotype is known, some institutions routinely provide red cells matched for Kell antigens to women (130). More comprehensive matching strategies for C/c, E/e, and K in women of childbearing age have shown that the success of such protocols is hindered by movement between regions with and without matching regimens (70). Despite this, Oud and colleagues recently showed that C/c, E/e, and K matching guidelines could significantly reduce rates of transfusion-associated alloimmunization in women when implemented systematically (131). Women undergoing IUT for alloimmune-mediated HDFN are at high risk for further sensitization. Several studies have demonstrated that up to a quarter of women will form new alloantibodies after IUT (132,133). Both fetal antigen exposure and exposure to antigens on transfused red cells pose a risk of maternal sensitization. Extended antigen matching for C/c, E/e, K, and Duffy, Kidd, and S antigens reduced the risk of alloimmunization after IUT by 60% in one study (134). Providing extended red cell matching in these settings requires a large inventory of phenotype or genotyped red cells. Building these inventories requires blood bank resources and diverse donor pools. Such efforts are currently limited by the lack of national transfusion and donor registries and have yet to be demonstrated cost-effective. While significant progress has been made in reducing the rates and mortality of HDFN in middle and high-income countries in the last 50 years, additional efforts are required to decrease rates of non-RhD HDFN and to ensure that all women worldwide have access to routine immunoprophylaxis and adequately matched red cell transfusions.
OH
OH is the leading cause of maternal morbidity and mortality in the United States and worldwide. OH is challenging to diagnose because of inaccurate estimation of blood loss by health practitioners. OH can be categorized as antepartum or PPH.
Antepartum hemorrhage (APH)
OH can occur during the antepartum and postpartum periods. Common causes of APH include placenta previa, placental abruption, and localized vulvar, vaginal, or cervical bleeding. APH affects 3–5% of pregnancies worldwide. According to the Royal College of Obstetricians and Gynecologists (RCOG), there is no consistent definition of APH (135). There are many risk factors for APH, including a previous cesarean section, smoking, drug abuse, multiparity, and advanced maternal age (136,137). Despite understanding the APH risk factors, prevention or prediction of APH is difficult (135). The management of APH includes a single course of antenatal corticosteroids to women between 24 and 32 weeks of gestation if preterm birth is anticipated, immediate delivery if fetal compromise is suspected, and anticipation of PPH in women with APH (135). In addition, utilization of locally created MTPs for APH is suggested. There are currently limited available data on blood product utilization and outcomes in APH, and additional studies are required.
PPH
PPH is a leading cause of maternal death worldwide (138). According to ACOG, PPH is defined as a total blood loss of 1,000 mL or greater or blood loss associated with signs and symptoms of hypovolemia that occurs within 24 hours of delivery (139). In contrast, the WHO defines PPH as blood loss of 500 mL or greater occurring within 24 hours of giving birth (140). ACOG has also recommended using a hematocrit decline of ≥10%, need for blood transfusion, and hemodynamic instability as other diagnostic criteria of PPH. PPH can be categorized as early PPH that occurs within 24 hours of delivery or late PPH that occurs after 24 hours of delivery but within 6 weeks postpartum (141). The incidence of PPH ranges from 4% to 10.8% based on the evaluation criteria utilized (142). Uterine atony and trauma, including trauma secondary to iatrogenic factors such as obstetric lacerations, are the primary causes of PPH (142). Other causes of PPH include inherited or acquired coagulopathies, uterine inversion, retained products of conception, abnormal placentation, genital tract trauma, and antepartum-induced PPH.
Similar to APH, the management of PPH requires a multidisciplinary hemorrhage protocol that is developed and implemented locally. The team should include anesthesiologists, obstetricians, and transfusion medicine physicians that work collaboratively to determine the underlying cause of bleeding, provide targeted treatments to control the bleeding, and supply blood transfusions. While risk factor stratification in PPH has not been demonstrated to be clinically useful, resources, including blood products and care, can be mobilized and escalated if at-risk women are identified early (143).
In observational study of 66,369 peripartum women, 1,540 women received a transfusion (144). As compared to women who were transfused with whole blood, pregnant women transfused with red cells had higher frequency of acute tubular necrosis. In contrast, in the same study, pulmonary edema was significantly increased in women who received only whole blood. Recommendations cannot be provided on the use of whole blood versus component therapy. There are currently no prospective randomized controlled clinical trials assessing the use of whole blood in women with OH. PPH is one of the most common indications for transfusions in sub-Saharan Africa and many low-resources regions (145). Access to a safe blood supply, either in the form of whole blood or component therapy, is mandatory to support women’s health worldwide.
MTPs
A significant complication of PPH is acute coagulopathy secondary to massive hemorrhage. MTPs in this population are based on those employed in non-obstetric patients. For patients undergoing massive transfusion for bleeding at rates that preclude the use of laboratory parameters to guide component therapy, many protocols utilize a balanced 1:1:1 ratio of plasma, platelets, and red cells to approximate whole blood. Some studies demonstrated a reduction in mortality in civilian and military trauma settings using a 1:1:1 ratio of blood products (146). The PROPPR randomized controlled trial compared utilization of a 1:1:1 versus a 1:1:2 blood product ratio in patients with trauma and major bleeding (147). There was no significant difference in mortality at 24 hours or 30 days in patients who received resuscitation with either a 1:1:1 or 1:1:2 blood product ratio; however, there were more deaths due to exsanguination in the 1:1:2 group. It is important to note, however, that these studies did not include patients with OH, and there is a lack of literature on optimal blood product ratios in pregnant women. Given this, there are no well-formulated recommendations on the appropriate ratio of blood products during obstetric-specific MTPs.
Cell salvage
Cell salvage use in the massively bleeding obstetric patient is increasing. While the use of cell salvage in pregnant women was initially controversial, improved filter systems that reduce the risk of amniotic fluid embolism in cell salvage instruments have enabled greater use of cell salvage in OH (148). The cell SALVage in Obstetrics (SALVO) study demonstrated that cell salvage use reduced the need for blood transfusions but was associated with increased exposure to fetal blood by the mother (149). If cell salvage is employed, the patient should undergo screening with a KB test to ensure adequate dosing of RhIG (150). Several guidelines recommend the use of cell salvage in women at high risk of PPH (150,151).
Antifibrinolytic therapy
Tranexamic acid (TXA) is an antifibrinolytic lysine analogue that competitively inhibits plasminogen activation. Antifibrinolytics are frequently utilized in the management of menorrhagia (152). As alterations in fibrinolysis have been implicated in the pathogenesis of PPH (153), there have been a number of studies exploring the use of TXA to treat and prevent PPH over the past several decades. The World Maternal Antifibrinolytic (WOMAN) trial is a seminal clinical trial that assessed the effect of TXA on PPH (154). In the WOMAN trial, 20,060 women with PPH at 193 hospitals in 21 different countries were randomized to receive either 1 to 2 grams of IV TXA or a placebo. Death due to bleeding was statistically significantly reduced in participants randomized to TXA. However, the rates of transfusion, hysterectomy, and surgical interventions were no different between the treatment groups. Adverse outcomes, including rates of venous thromboembolism and renal dysfunction, were not increased in the TXA arm. Notably, the benefit of TXA at reducing PPH was most apparent when treatment was initiated within 1–3 hours of delivery. A subsequent Cochrane review also supported the use of TXA in treating PPH (155). In response to the publication of the WOMAN trial, the WHO updated guidelines to strongly recommend early use of TXA in PPH in women delivering both vaginally as well as via Cesarean section (156).
As most study sites in the WOMAN trial were in low-income areas, questions regarding whether the results are generalizable to more well-resourced countries have been raised (157). Sudhof and colleagues (158) performed a cost-effectiveness analysis which supported that TXA is likely to be cost-saving even in the United States. Given the positive benefit to risk ratio, most obstetric guidelines recommend the use of TXA in combination with surgical and medical measures to treat PPH (151,156).
The Tranexamic Acid for Preventing Postpartum Hemorrhage Following a Vaginal Delivery (TRAAP) trial was a large, multicenter randomized, controlled trial that examined whether 1 gram of TXA provided concomitantly with prophylactic oxytocin reduced PPH (159). Over 4,000 study participants were enrolled in the trial. PPH occurred in 8.1% of women randomized to the TXA group compared to 9.8% of the placebo. This difference did not reach statistical significance; however a subgroup analysis showed that for women with operative vaginal deliveries the rates of PPH were lower in the TXA arm. More recently, the TRAAP2 trial assessed the efficacy of TXA at preventing PPH in women undergoing cesarean deliveries (160). Similar to the TRAAP study, slightly over 4,000 women undergoing cesarean deliveries were randomized to receive placebo or 1 gram of IV TXA. PPH, defined as receiving a red cell transfusion within 2 days of delivery or calculated estimated blood loss greater than 1,000 mL, occurred in 26.7% of the TXA group and 31.6% of the placebo group (P=0.003). There was no significant difference, however, in provider-assessed clinically significant PPH. Additional trials utilizing clinically relevant endpoints are required to understand the role of antifibrinolytics for prophylaxis rather than treatment of PPH.
Populations with unique transfusion requirements in pregnancy
Transfusion therapy for SCD in pregnancy
SCD is an inherited hemoglobinopathy defined by the presence of biallelic abnormal β-globin genes (hemoglobin SS, SC, or Sβ thalassemia). Due to improvements in the diagnosis, treatment, and care of patients with SCD, more patients with SCD are surviving to reproductive age. Women with SCD have a higher risk of pregnancy-associated morbidity and obstetric complications such as PEC, venothromboembolism, placental abruption, intrauterine growth restriction, and preterm birth (161,162). Both maternal and perinatal mortality rates are significantly higher in SCD as compared to women without SCD (163,164). Pregnant women with SCD are also more likely to experience SCD complications, including acute chest syndrome, veno-occlusive episodes (VOE), and pulmonary hypertension (165).
Animal studies have demonstrated hydroxyurea to be teratogenic (166). As such, there are limited options for disease-modifying therapy aside from red cell transfusions in pregnant women with SCD. For patients receiving antenatal chronic transfusion therapy, for primary or secondary stroke prophylaxis, for example, it is recommended that transfusion therapy continues during pregnancy. Whether chronic transfusion therapy should be initiated prophylactically in all pregnant women with SCD is controversial and remains an area of active investigation (167). There is a single published randomized trial comparing scheduled, prophylactic to on-demand transfusion therapy (168). Prophylactic transfusion significantly reduced the incidence of VOE during pregnancy, though there was no difference in fetal outcomes. A recent meta-analysis of 12 observational studies of chronic transfusion in pregnant women with SCD showed a reduction in pain episodes, pulmonary complications, pulmonary embolism, and maternal mortality; however, the overall quality of evidence was judged to be low (169). Current evidence supports either prophylactic transfusion or transfusion on demand for anemia or complications in pregnant women with SCD (169). Additional well-designed, large trials are required.
Individualized transfusion plans, including transfusion method, indications, and goals, should be established early in pregnancy for all women with SCD (170). As many patients with SCD receive red cell transfusions at multiple institutions, it is important to gather transfusion and red cell antibody histories from all locations where a patient has been previously transfused, particularly as many red cell alloantibodies are evanescent (171). Prior to the first transfusion, an extended red cell antigen profile for C/c, E/e, K/k, Jka/Jkb, Fya/Fyb, M/N, and S/s should be obtained (169). Red cell transfusions should be matched for C, E, and K antigens to reduce rates of red cell alloimmunization (169). For patients with extensive red cell alloimmunization or a history of delayed hemolytic transfusion reactions, extended antigen matching should be considered.
The potential benefits of red cell transfusion during pregnancy in patients with SCD must be weighed against the risks of transfusion-transmitted infections, iron overload, red cell alloimmunization, and transfusion reactions. Red cell alloimmunization can lead to HDFN and increases the risk of delayed hemolytic transfusion reactions, a potential source of severe, life-threatening anemia in patients with SCD. As part of a multidisciplinary care team, transfusion medicine specialists can play critical roles in the management of pregnancy in SCD by assessing potential adverse risks of transfusion and guiding the selection of red cells.
Management of VWD in pregnancy
VWD is the most common inherited bleeding disorder with a prevalence of approximately 1% (172,173). Type 1 and type 3 VWD are characterized by quantitative deficiencies in von Willebrand factor (VWF), while type 2 VWD, which encompasses types 2A, 2B, 2N, and 2M, results from qualitative defects in VWF. The levels of VWF and factor VIII physiologically increase throughout pregnancy as part of the body’s hemostatic shift toward procoagulation in preparation for delivery-related blood loss (174,175). VWF levels also increase in pregnant women with types 1 and 2 VWD, often peaking at levels 200–300% higher than baseline (176,177). It is important to note, however, that while VWF antigen levels increase in pregnant patients with type 2 VWD, functional VWF activity remains low. In pregnant women with severe deficiencies in VWF (type 3 VWD), VWF and factor VIII levels do not achieve similar increases (178).
While the majority of patients with VWD normalize VWF levels and activity peripartum, women with VWD suffer higher rates of both antepartum bleeding as well as PPH (179). Women with VWD are also at increased risk of delayed PPH, occurring several weeks after delivery when VWF levels decline to baseline. Expert consensus guidelines recommend measurement of VWF and factor VIII before antenatal procedures such as chorionic villus sampling and amniocentesis and for all women with VWD in the third trimester (180-182). Despite these recommendations, a recent study showed that only one-third of pregnant women with VWD in the United States have third-trimester VWF measurements, and women who did not undergo VWF monitoring had significantly higher rates of PPH as compared to those with recorded third-trimester VWF levels (183).
There are limited controlled trials assessing factor replacement in pregnant women with VWD. Most guidelines recommend the use of desmopressin or VWF concentrate when VWF levels measure <50 IU/dL with bleeding, at delivery, or before providing neuraxial anesthesia (180-182). Desmopressin stimulates endothelial cells to synthesize and release VWF, but potential adverse effects include seizures, hyponatremia, and hypotension. Desmopressin should be utilized only for patients who have previously shown a response with a desmopressin challenge, and some guidelines recommend limiting use to the first and second trimesters. VWF is available in both recombinant and plasma-derived formulations, which are typically dosed at delivery and for several days postpartum. A single small study suggests that recombinant and plasma-derived VWF have similar efficacy in the prevention and treatment of PPH in VWD; however, additional larger controlled trials are required (184).
Recent studies have revealed that patients with VWD have higher rates of PPH even when receiving therapy to maintain VWF >50 IU/dL (185,186). Accordingly, some experts now recommend treatment for VWF levels <80 IU/dL in pregnant women with VWD (178). There are several ongoing clinical trials examining rates of PPH when higher levels of VWF are maintained in pregnant women (178,187). The results of these investigations and implementation of universal VWF testing in the third trimester are expected to improve and optimize care for women with VWD.
Other inherited bleeding disorders in pregnancy
Hemophilia A and B are X-linked hereditary bleeding disorders due to deficiencies of factors VIII and IX, respectively. Female hemophilia carriers typically have low baseline factor levels, approximately 50% of normal; however, some hemophilia carriers with extreme lyonization have unexpectedly low factor levels and more severe bleeding diathesis (188). Factor VIII levels physiologically increase during pregnancy and often normalize in hemophilia A carriers. Factor IX does not increase significantly in pregnancy. While large studies examining bleeding risks in hemophilia carriers during pregnancy are lacking, observational cohort studies support that hemophilia carriers are at increased risk for both primary and secondary PPH (189,190). Whether hemophilia carriers have higher rates of miscarriage and antepartum bleeding requires further investigation.
Factor levels should be monitored at baseline and at 28 and 34 weeks gestation and before invasive procedures during pregnancy in hemophilia carriers. Most guidelines recommend factor replacement at delivery when factor VIII or IX levels are below 50 IU/dL in the third trimester (178,191,192). Factor VIII and IX concentrates are considered first-line agents for peripartum prophylaxis in hemophilia carriers with low factor levels. Desmopressin has been reported as an alternative treatment in hemophilia A carriers, though concerns have been raised regarding the safety of desmopressin use during the third trimester (193). Despite peripartum factor replacement to maintain levels ≥50 IU/dL, as noted above, some studies suggest that hemophilia A carriers remain at higher risk of PPH, likely because physiological factor VIII levels are 100–200% higher than baseline in normal women at the time of delivery (190,194). An ongoing clinical trial investigates whether a factor VIII and IX cutoff value of 80 IU/dL for treatment with factor concentrates reduces PPH in hemophilia carriers (Prides study; NTR6947).
Women with rare inherited bleeding disorders, including deficiencies of factor II, V, VII, X, XI, XIII, and congenital fibrinogen disorders, are also at higher risk of bleeding and pregnancy complications. While factors VII, X, XII, and fibrinogen increase during pregnancy, factor XIII levels decrease (195). Women with inherited factor XIII and fibrinogen deficiencies have higher rates of miscarriage and placental abnormalities and require factor replacement early in pregnancy to prevent pregnancy loss (196,197). Compared to hemophilia carriers and women with VWD, antepartum bleeding is more common in patients with factor V and X deficiencies. There are limited data regarding factor replacement and care of women with rare inherited bleeding disorders during pregnancy and delivery, as publications are primarily limited to case reports and small case series. When available, factor concentrates, and prothrombin complex concentrates are preferred over FFP or cryoprecipitate (Table 1). Most guidelines recommend replacement prophylaxis at delivery and for 2–4 days postpartum; however, additional, well-designed studies are required to better understand optimal factor levels and dosing schedules.
Table 1
Bleeding disorders | Factor replacement agents | Adjunct agents to prevent or treat bleeding |
---|---|---|
Fibrinogen disorders | Plasma-derived fibrinogen concentrates | Antifibrinolytics |
Cryoprecipitate | ||
Factor II deficiency | Prothrombin complex concentrates | Antifibrinolytics |
FFP | ||
Factor V deficiency | FFP | Antifibrinolytics |
Factor VII deficiency | Recombinant factor VIIa | Antifibrinolytics |
Prothrombin complex concentrates | ||
FFP | ||
Hemophilia A carrier (factor VIII deficiency) | Recombinant factor VIII | Antifibrinolytics |
Plasma-derived factor VIII concentrates | Desmopressin | |
Hemophilia B carrier (factor IX deficiency) | Recombinant factor IX | Antifibrinolytics |
Plasma-derived factor IX concentrates | ||
Factor X deficiency | Prothrombin complex concentrate | Antifibrinolytics |
FFP | ||
Factor XI deficiency | Plasma-derived factor XI concentrates* | Antifibrinolytics |
FFP | ||
Factor XIII deficiency | Plasma-derived factor XIII concentrates | Antifibrinolytics |
Cryoprecipitate | ||
FFP |
When available, factor concentrates and recombinant factors are generally preferred over cryoprecipitate and FFP, given the reduced risk of transfusion reactions and transfusion transmitted infections. Antifibrinolytics such as tranexamic acid can be used as adjuncts to reduce postpartum hemorrhage. *, not available in the United States. FFP, fresh frozen plasma.
Conclusions
While advances such as RhIG, IUT techniques, and phototherapy have reduced the rates of adverse outcomes secondary to RhD and ABO HDFN over the last 50 years, many of these tools are not widely available in low-income countries. Alloimmunization to other red cell antigens continues to pose challenges, and additional technologies must be developed to mitigate alloimmunization secondary to transfusion and pregnancy. Deaths secondary to antepartum and PPH remain high worldwide. Efforts to expand access to a safe blood supply and adjunct therapies, including iron and antifibrinolytics, are mandatory to eliminate preventable maternal deaths. The transfusion medicine specialist can provide a critical role in caring for the hematologic conditions of pregnancy and advancing efforts to support equal access to lifesaving transfusion therapy globally.
Acknowledgments
We want to thank Amanda Shanahan, BSN, RN who helped with the figure in this manuscript. The table and figure are original and created by Dr. Grace E. Linder.
Funding: None.
Footnote
Provenance and Peer Review: This article was commissioned by the Guest Editor (Paul D. Mintz) for the series “Transfusion Therapy: Principles and Practices” published in Annals of Blood. The article has undergone external peer review.
Conflicts of Interest: Both authors have completed the ICMJE uniform disclosure form (available at https://aob.amegroups.com/article/view/10.21037/aob-21-73/coif). The series “Transfusion Therapy: Principles and Practices” was commissioned by the editorial office without any funding or sponsorship. TSI is a consultant for Terumo Blood and Cell Technologies. The authors have no other conflicts of interest to declare.
Ethical Statement: The authors are accountable for all aspects of the work in ensuring that questions related to the accuracy or integrity of any part of the work are appropriately investigated and resolved.
Open Access Statement: This is an Open Access article distributed in accordance with the Creative Commons Attribution-NonCommercial-NoDerivs 4.0 International License (CC BY-NC-ND 4.0), which permits the non-commercial replication and distribution of the article with the strict proviso that no changes or edits are made and the original work is properly cited (including links to both the formal publication through the relevant DOI and the license). See: https://creativecommons.org/licenses/by-nc-nd/4.0/.
References
- Bruinse HW, van den Berg H, Haspels AA. Smoking and its effect on maternal plasma volume during and after normal pregnancy. Eur J Obstet Gynecol Reprod Biol 1985;20:215-9. [Crossref] [PubMed]
- Aguree S, Gernand AD. Plasma volume expansion across healthy pregnancy: a systematic review and meta-analysis of longitudinal studies. BMC Pregnancy Childbirth 2019;19:508. [Crossref] [PubMed]
- de Haas S, Ghossein-Doha C, van Kuijk SM, et al. Physiological adaptation of maternal plasma volume during pregnancy: a systematic review and meta-analysis. Ultrasound Obstet Gynecol 2017;49:177-87. [Crossref] [PubMed]
- Pivarnik JM, Mauer MB, Ayres NA, et al. Effects of chronic exercise on blood volume expansion and hematologic indices during pregnancy. Obstet Gynecol 1994;83:265-9. [PubMed]
- Gibson HM. Plasma volume and glomerular filtration rate in pregnancy and their relation to differences in fetal growth. J Obstet Gynaecol Br Commonw 1973;80:1067-74. [Crossref] [PubMed]
- Thomsen JK, Fogh-Andersen N, Jaszczak P. Atrial natriuretic peptide, blood volume, aldosterone, and sodium excretion during twin pregnancy. Acta Obstet Gynecol Scand 1994;73:14-20. [Crossref] [PubMed]
- West CA, Sasser JM, Baylis C. The enigma of continual plasma volume expansion in pregnancy: critical role of the renin-angiotensin-aldosterone system. Am J Physiol Renal Physiol 2016;311:F1125-34. [Crossref] [PubMed]
- Pirani BB, Campbell DM, MacGillivray I. Plasma volume in normal first pregnancy. J Obstet Gynaecol Br Commonw 1973;80:884-7. [Crossref] [PubMed]
- Goodlin RC, Dobry CA, Anderson JC, et al. Clinical signs of normal plasma volume expansion during pregnancy. Am J Obstet Gynecol 1983;145:1001-9. [Crossref] [PubMed]
- Scholten RR, Sep S, Peeters L, et al. Prepregnancy low-plasma volume and predisposition to preeclampsia and fetal growth restriction. Obstet Gynecol 2011;117:1085-93. [Crossref] [PubMed]
- Vonck S, Staelens AS, Lanssens D, et al. Low volume circulation in normotensive women pregnant with neonates small for gestational age. Fetal Diagn Ther 2019;46:238-45. [Crossref] [PubMed]
- Salas SP, Rosso P, Espinoza R, et al. Maternal plasma volume expansion and hormonal changes in women with idiopathic fetal growth retardation. Obstet Gynecol 1993;81:1029-33. [PubMed]
- Donckers J, Scholten RR, Oyen WJ, et al. Unexplained first trimester recurrent pregnancy loss and low venous reserves. Hum Reprod 2012;27:2613-8. [Crossref] [PubMed]
- Ouzounian JG, Elkayam U. Physiologic changes during normal pregnancy and delivery. Cardiol Clin 2012;30:317-29. [Crossref] [PubMed]
- Heslehurst N, Rankin J, Wilkinson JR, et al. A nationally representative study of maternal obesity in England, UK: trends in incidence and demographic inequalities in 619 323 births, 1989-2007. Int J Obes (Lond) 2010;34:420-8. [Crossref] [PubMed]
- Fuchs F, Monet B, Ducruet T, et al. Effect of maternal age on the risk of preterm birth: A large cohort study. PLoS One 2018;13:e0191002. [Crossref] [PubMed]
- Chesley LC. Plasma and red cell volumes during pregnancy. Am J Obstet Gynecol 1972;112:440-50. [Crossref] [PubMed]
- Milman N, Byg KE, Agger AO. Hemoglobin and erythrocyte indices during normal pregnancy and postpartum in 206 women with and without iron supplementation. Acta Obstet Gynecol Scand 2000;79:89-98. [Crossref] [PubMed]
- Pavord S, Daru J, Prasannan N, et al. UK guidelines on the management of iron deficiency in pregnancy. Br J Haematol 2020;188:819-30. [Crossref] [PubMed]
- Achebe MM, Gafter-Gvili A. How I treat anemia in pregnancy: iron, cobalamin, and folate. Blood 2017;129:940-9. [Crossref] [PubMed]
- Organization WH. Haemoglobin concentrations for the diagnosis of anaemia and assessment of severity. World Health Organization 2011.
- De Benoist B, Cogswell M, Egli I, et al. Worldwide prevalence of anaemia 1993-2005. WHO Global Database of anaemia 2008.
- Nair M, Churchill D, Robinson S, et al. Association between maternal haemoglobin and stillbirth: a cohort study among a multi-ethnic population in England. Br J Haematol 2017;179:829-37. [Crossref] [PubMed]
- Bothwell TH. Iron requirements in pregnancy and strategies to meet them. Am J Clin Nutr 2000;72:257S-64S. [Crossref] [PubMed]
- Roy NBA, Pavord S. The management of anaemia and haematinic deficiencies in pregnancy and post-partum. Transfus Med 2018;28:107-16. [Crossref] [PubMed]
- Organization WH. Nutritional anaemias: tools for effective prevention and control. World Health Organization 2017:1-83.
- Wassef A, Nguyen QD, St-André M. Anaemia and depletion of iron stores as risk factors for postpartum depression: a literature review. J Psychosom Obstet Gynaecol 2019;40:19-28. [Crossref] [PubMed]
- Briley A, Seed PT, Tydeman G, et al. Reporting errors, incidence and risk factors for postpartum haemorrhage and progression to severe PPH: a prospective observational study. BJOG 2014;121:876-88. [Crossref] [PubMed]
- Rahman MM, Abe SK, Rahman MS, et al. Maternal anemia and risk of adverse birth and health outcomes in low- and middle-income countries: systematic review and meta-analysis. Am J Clin Nutr 2016;103:495-504. [Crossref] [PubMed]
- Lone FW, Qureshi RN, Emanuel F. Maternal anaemia and its impact on perinatal outcome. Trop Med Int Health 2004;9:486-90. [Crossref] [PubMed]
- Congdon EL, Westerlund A, Algarin CR, et al. Iron deficiency in infancy is associated with altered neural correlates of recognition memory at 10 years. J Pediatr 2012;160:1027-33. [Crossref] [PubMed]
- Veena SR, Gale CR, Krishnaveni GV, et al. Association between maternal nutritional status in pregnancy and offspring cognitive function during childhood and adolescence; a systematic review. BMC Pregnancy Childbirth 2016;16:220. [Crossref] [PubMed]
- Zaman B, Rasool S, Jasim S, et al. Hepcidin as a diagnostic biomarker of iron deficiency anemia during pregnancy. J Matern Fetal Neonatal Med 2021;34:1288-96. [Crossref] [PubMed]
- Bah A, Pasricha SR, Jallow MW, et al. Serum hepcidin concentrations decline during pregnancy and may identify iron deficiency: analysis of a longitudinal pregnancy cohort in the Gambia. J Nutr 2017;147:1131-7. [Crossref] [PubMed]
- Breymann C, Milman N, Mezzacasa A, et al. Ferric carboxymaltose vs. oral iron in the treatment of pregnant women with iron deficiency anemia: an international, open-label, randomized controlled trial (FER-ASAP). J Perinat Med 2017;45:443-53. [Crossref] [PubMed]
- Kochhar PK, Kaundal A, Ghosh P. Intravenous iron sucrose versus oral iron in treatment of iron deficiency anemia in pregnancy: a randomized clinical trial. J Obstet Gynaecol Res 2013;39:504-10. [Crossref] [PubMed]
- Abraha I, Bonacini MI, Montedori A, et al. Oral iron-based interventions for prevention of critical outcomes in pregnancy and postnatal care: An overview and update of systematic reviews. J Evid Based Med 2019;12:155-66. [Crossref] [PubMed]
- VanderMeulen H, Strauss R, Lin Y, et al. The contribution of iron deficiency to the risk of peripartum transfusion: a retrospective case control study. BMC Pregnancy Childbirth 2020;20:196. [Crossref] [PubMed]
- Burrows RF, Kelton JG. Fetal thrombocytopenia and its relation to maternal thrombocytopenia. N Engl J Med 1993;329:1463-6. [Crossref] [PubMed]
- Boehlen F, Hohlfeld P, Extermann P, et al. Platelet count at term pregnancy: a reappraisal of the threshold. Obstet Gynecol 2000;95:29-33. [Crossref] [PubMed]
- Gernsheimer T, James AH, Stasi R. How I treat thrombocytopenia in pregnancy. Blood 2013;121:38-47. [Crossref] [PubMed]
- ACOG Practice Bulletin No. 207: thrombocytopenia in pregnancy. Obstet Gynecol 2019;133:e181-93. [Crossref] [PubMed]
- Cines DB, Levine LD. Thrombocytopenia in pregnancy. Hematology Am Soc Hematol Educ Program 2017;2017:144-51. [Crossref] [PubMed]
- Fogerty AE, Dzik W. Gestational thrombocytopenia: a case-control study of over 3,500 pregnancies. Br J Haematol 2021;194:433-8. [Crossref] [PubMed]
- Rottenstreich A, Israeli N, Roth B, et al. Risk factors associated with neonatal thrombocytopenia in pregnant women with immune thrombocytopenic purpura. J Matern Fetal Neonatal Med 2020;33:1572-8. [Crossref] [PubMed]
- Otrock ZK, Liu C, Grossman BJ. Platelet transfusion in thrombotic thrombocytopenic purpura. Vox Sang 2015;109:168-72. [Crossref] [PubMed]
- Clements T, Rice TF, Vamvakas G, et al. Update on transplacental transfer of IgG subclasses: impact of maternal and fetal factors. Front Immunol 2020;11:1920. [Crossref] [PubMed]
- Gilby DM, Mee JB, Kamlin COF, et al. Outcomes following antenatal identification of hydrops fetalis: a single-centre experience from 2001 to 2012. Arch Dis Child Fetal Neonatal Ed 2019;104:F253-8. [Crossref] [PubMed]
- Bhatt DK, Mehrotra A, Gaedigk A, et al. Age- and genotype-dependent variability in the protein abundance and activity of six major uridine diphosphate-glucuronosyltransferases in human liver. Clin Pharmacol Ther 2019;105:131-41. [Crossref] [PubMed]
- Bhutani VK, Wong RJ, Stevenson DK. Hyperbilirubinemia in preterm neonates. Clin Perinatol 2016;43:215-32. [Crossref] [PubMed]
- Alkén J, Håkansson S, Ekéus C, et al. Rates of extreme neonatal hyperbilirubinemia and kernicterus in children and adherence to national guidelines for screening, diagnosis, and treatment in Sweden. JAMA Netw Open 2019;2:e190858. [Crossref] [PubMed]
- Jackson ME, Baker JM. Hemolytic Disease of the Fetus and Newborn: Historical and Current State. Clin Lab Med 2021;41:133-51. [Crossref] [PubMed]
- Hendrickson JE, Delaney M. Hemolytic disease of the fetus and newborn: modern practice and future investigations. Transfus Med Rev 2016;30:159-64. [Crossref] [PubMed]
- Miller DF, Petrie SJ. Fatal erythroblastosis fetalis secondary to ABO Incompatibility. Report of a case. Obstet Gynecol 1963;22:773-7. [PubMed]
- Klein HG, Anstee DJ. Mollison’s blood transfusion in clinical medicine. 12th edition. Chichester: Wiley Blackwell, 2014.
- Voak D, Williams MA. An explanation of the failure of the direct antiglobulin test to detect erythrocyte sensitization in ABO haemolytic disease of the newborn and observations on pinocytosis of IgG anti-A antibodies by infant (cord) red cells. Br J Haematol 1971;20:9-23. [Crossref] [PubMed]
- Voak D, Bowley CC. A detailed serological study on the prediction and diagnosis of ABO haemolytic disease of the newborn (ABO HD). Vox Sang 1969;17:321-48. [Crossref] [PubMed]
- Krog GR, Donneborg ML, Hansen BM, et al. Prediction of ABO hemolytic disease of the newborn using pre- and perinatal quantification of maternal anti-A/anti-B IgG titer. Pediatr Res 2021;90:74-81. [Crossref] [PubMed]
- Moise KJ. Hemolytic disease of the fetus and newborn. Clin Adv Hematol Oncol 2013;11:664-6. [PubMed]
- Reid M, Lomas-Francis C, Olsson M. The blood group antigen factsbook. 3rd Edition. Academic press, 2012.
- Ree IMC, Smits-Wintjens VEHJ, van der Bom JG, et al. Neonatal management and outcome in alloimmune hemolytic disease. Expert Rev Hematol 2017;10:607-16. [Crossref] [PubMed]
- Osaro E, Charles AT. Rh isoimmunization in Sub-Saharan Africa indicates need for universal access to anti-RhD immunoglobulin and effective management of D-negative pregnancies. Int J Womens Health 2010;2:429-37. [Crossref] [PubMed]
- Ayenew AA. Prevalence of rhesus D-negative blood type and the challenges of rhesus D immunoprophylaxis among obstetric population in Ethiopia: a systematic review and meta-analysis. Matern Health Neonatol Perinatol 2021;7:8. [Crossref] [PubMed]
- Cohn CS, Delaney, M, Johnson ST, et al. editor(s). Technical Manual. 20th edition. Bethesda: AABB Press, 2020.
- Pollock JM, Bowman JM. Anti-Rh(D) IgG subclasses and severity of Rh hemolytic disease of the newborn. Vox Sang 1990;59:176-9. [Crossref] [PubMed]
- Lambin P, Debbia M, Puillandre P, et al. IgG1 and IgG3 anti-D in maternal serum and on the RBCs of infants suffering from HDN: relationship with the severity of the disease. Transfusion 2002;42:1537-46. [Crossref] [PubMed]
- Kapur R, Della Valle L, Sonneveld M, et al. Low anti-RhD IgG-Fc-fucosylation in pregnancy: a new variable predicting severity in haemolytic disease of the fetus and newborn. Br J Haematol 2014;166:936-45. [Crossref] [PubMed]
- Sonneveld ME, Koelewijn J, de Haas M, et al. Antigen specificity determines anti-red blood cell IgG-Fc alloantibody glycosylation and thereby severity of haemolytic disease of the fetus and newborn. Br J Haematol 2017;176:651-60. [Crossref] [PubMed]
- Maier JT, Schalinski E, Schneider W, et al. Fetomaternal hemorrhage (FMH), an update: review of literature and an illustrative case. Arch Gynecol Obstet 2015;292:595-602. [Crossref] [PubMed]
- Delaney M, Wikman A, van de Watering L, et al. Blood Group Antigen Matching Influence on Gestational Outcomes (AMIGO) study. Transfusion 2017;57:525-32. [Crossref] [PubMed]
- Slootweg YM, Koelewijn JM, van Kamp IL, et al. Third trimester screening for alloimmunisation in Rhc-negative pregnant women: evaluation of the Dutch national screening programme. BJOG 2016;123:955-63. [Crossref] [PubMed]
- Levine P. Serological factors as possible causes in spontaneous abortions. In: Rhesus haemolytic disease. Dordrecht: Springer, 1943.
- Webb J, Delaney M. Red blood cell alloimmunization in the pregnant patient. Transfus Med Rev 2018;32:213-9. [Crossref] [PubMed]
- Zwiers C, Koelewijn JM, Vermij L, et al. ABO incompatibility and RhIG immunoprophylaxis protect against non-D alloimmunization by pregnancy. Transfusion 2018;58:1611-7. [Crossref] [PubMed]
- Koelewijn JM, Vrijkotte TG, de Haas M, et al. Risk factors for the presence of non-rhesus D red blood cell antibodies in pregnancy. BJOG 2009;116:655-64. [Crossref] [PubMed]
- Rahimi-Levene N, Chezar J, Yahalom V, et al. Red blood cell alloimmunization prevalence and hemolytic disease of the fetus and newborn in Israel: a retrospective study. Transfusion 2020;60:2684-90. [Crossref] [PubMed]
- Awowole I, Cohen K, Rock J, et al. Prevalence and obstetric outcome of women with red cell antibodies in pregnancy at the Leeds Teaching Hospitals NHS Trust, West Yorkshire, England. Eur J Obstet Gynecol Reprod Biol 2019;237:89-92. [Crossref] [PubMed]
- Das S, Shastry S, Rai L, et al. Frequency and clinical significance of red cell antibodies in pregnancy - A prospective study from India. Indian J Pathol Microbiol 2020;63:241-6. [Crossref] [PubMed]
- ACOG practice bulletin No. 192: management of alloimmunization during pregnancy. Obstet Gynecol 2018;131:e82-90. [Crossref] [PubMed]
- White J, Qureshi H, Massey E, et al. Guideline for blood grouping and red cell antibody testing in pregnancy. Transfus Med 2016;26:246-63. [Crossref] [PubMed]
- Alfirevic Z, Navaratnam K, Mujezinovic F. Amniocentesis and chorionic villus sampling for prenatal diagnosis. Cochrane Database Syst Rev 2017;9:CD003252. [Crossref] [PubMed]
- Daniels G, Finning K, Lozano M, et al. Vox Sanguinis International Forum on application of fetal blood grouping: summary. Vox Sang 2018;113:198-201. [Crossref] [PubMed]
- Haimila K, Sulin K, Kuosmanen M, et al. Targeted antenatal anti-D prophylaxis program for RhD-negative pregnant women-outcome of the first two years of a national program in Finland. Acta Obstet Gynecol Scand 2017;96:1228-33. [Crossref] [PubMed]
- Clausen FB, Steffensen R, Christiansen M, et al. Routine noninvasive prenatal screening for fetal RHD in plasma of RhD-negative pregnant women-2years of screening experience from Denmark. Prenat Diagn 2014;34:1000-5. [Crossref] [PubMed]
- Scheffer PG, van der Schoot CE, Page-Christiaens GC, et al. Noninvasive fetal blood group genotyping of rhesus D, c, E and of K in alloimmunised pregnant women: evaluation of a 7-year clinical experience. BJOG 2011;118:1340-8. [Crossref] [PubMed]
- Lieberman L, Andrews J, Evans MD, et al. Comparison of prenatal anti-D titration testing by gel and tube methods: A review of the literature. Transfusion 2021;61:1749-56. [Crossref] [PubMed]
- Koelewijn JM, Slootweg YM, Folman C, et al. Diagnostic value of laboratory monitoring to predict severe hemolytic disease of the fetus and newborn in non-D and non-K-alloimmunized pregnancies. Transfusion 2020;60:391-9. [Crossref] [PubMed]
- Slootweg YM, Lindenburg IT, Koelewijn JM, et al. Predicting anti-Kell-mediated hemolytic disease of the fetus and newborn: diagnostic accuracy of laboratory management. Am J Obstet Gynecol 2018;219:393.e1-8. [Crossref] [PubMed]
- Bowman JM, Pollock JM. Amniotic fluid spectrophotometry and early delivery in the management of erythroblastosis fetalis. Pediatrics 1965;35:815-35. [Crossref] [PubMed]
- Mari G, Deter RL, Carpenter RL, et al. Noninvasive diagnosis by Doppler ultrasonography of fetal anemia due to maternal red-cell alloimmunization. Collaborative Group for Doppler Assessment of the Blood Velocity in Anemic Fetuses. N Engl J Med 2000;342:9-14. [Crossref] [PubMed]
- Zwiers C, van Kamp I, Oepkes D, et al. Intrauterine transfusion and non-invasive treatment options for hemolytic disease of the fetus and newborn - review on current management and outcome. Expert Rev Hematol 2017;10:337-44. [Crossref] [PubMed]
- Lindenburg IT, van Kamp IL, van Zwet EW, et al. Increased perinatal loss after intrauterine transfusion for alloimmune anaemia before 20 weeks of gestation. BJOG 2013;120:847-52. [Crossref] [PubMed]
- Zwiers C, van der Bom JG, van Kamp IL, et al. Postponing Early intrauterine Transfusion with Intravenous immunoglobulin Treatment; the PETIT study on severe hemolytic disease of the fetus and newborn. Am J Obstet Gynecol 2018;219:291.e1-291.e9. [Crossref] [PubMed]
- Connan K, Kornman L, Savoia H, et al. IVIG - is it the answer? Maternal administration of immunoglobulin for severe fetal red blood cell alloimmunisation during pregnancy: a case series. Aust N Z J Obstet Gynaecol 2009;49:612-8. [Crossref] [PubMed]
- Voto LS, Mathet ER, Zapaterio JL, et al. High-dose gammaglobulin (IVIG) followed by intrauterine transfusions (IUTs): a new alternative for the treatment of severe fetal hemolytic disease. J Perinat Med 1997;25:85-8. [Crossref] [PubMed]
- Barclay GR, Greiss MA, Urbaniak SJ. Adverse effect of plasma exchange on anti-D production in rhesus immunisation owing to removal of inhibitory factors. Br Med J 1980;280:1569-71. [Crossref] [PubMed]
- Lépine MS, Goua V, Debouverie OS, et al. Multidisciplinary management of anti-PP1Pk or anti-P alloimmunization during pregnancy: a new case with anti-P and a literature review. Transfusion 2021;61:1972-9. [Crossref] [PubMed]
- Ruma MS, Moise KJ Jr, Kim E, et al. Combined plasmapheresis and intravenous immune globulin for the treatment of severe maternal red cell alloimmunization. Am J Obstet Gynecol 2007;196:138.e1-6. [Crossref] [PubMed]
- Houston BL, Govia R, Abou-Setta AM, et al. Severe Rh alloimmunization and hemolytic disease of the fetus managed with plasmapheresis, intravenous immunoglobulin and intrauterine transfusion: a case report. Transfus Apher Sci 2015;53:399-402. [Crossref] [PubMed]
- Borghi S, Bournazos S, Thulin NK, et al. FcRn, but not FcγRs, drives maternal-fetal transplacental transport of human IgG antibodies. Proc Natl Acad Sci U S A 2020;117:12943-51. [Crossref] [PubMed]
- Chen P, Li C, Lang S, et al. Animal model of fetal and neonatal immune thrombocytopenia: role of neonatal Fc receptor in the pathogenesis and therapy. Blood 2010;116:3660-8. [Crossref] [PubMed]
- Castleman JS, Moise KJ Jr, Kilby MD. Medical therapy to attenuate fetal anaemia in severe maternal red cell alloimmunisation. Br J Haematol 2021;192:425-32. [Crossref] [PubMed]
- Gable KL, Guptill JT. Antagonism of the neonatal fc receptor as an emerging treatment for myasthenia gravis. Front Immunol 2020;10:3052. [Crossref] [PubMed]
- Hansen TWR, Maisels MJ, Ebbesen F, et al. Sixty years of phototherapy for neonatal jaundice - from serendipitous observation to standardized treatment and rescue for millions. J Perinatol 2020;40:180-93. [Crossref] [PubMed]
- Bhutani VK, Stark AR, Lazzeroni LC, et al. Predischarge screening for severe neonatal hyperbilirubinemia identifies infants who need phototherapy. J Pediatr 2013;162:477-482.e1. [Crossref] [PubMed]
- Steiner LA, Bizzarro MJ, Ehrenkranz RA, et al. A decline in the frequency of neonatal exchange transfusions and its effect on exchange-related morbidity and mortality. Pediatrics 2007;120:27-32. [Crossref] [PubMed]
- Chitty HE, Ziegler N, Savoia H, et al. Neonatal exchange transfusions in the 21st century: a single hospital study. J Paediatr Child Health 2013;49:825-32. [Crossref] [PubMed]
- Zwiers C, Scheffer-Rath ME, Lopriore E, et al. Immunoglobulin for alloimmune hemolytic disease in neonates. Cochrane Database Syst Rev 2018;3:CD003313. [Crossref] [PubMed]
- Santos MC, Sá C, Gomes SC Jr, et al. The efficacy of the use of intravenous human immunoglobulin in Brazilian newborns with rhesus hemolytic disease: a randomized double-blind trial. Transfusion 2013;53:777-82. [Crossref] [PubMed]
- Yang Y, Pan JJ, Zhou XG, et al. The effect of immunoglobulin treatment for hemolysis on the incidence of necrotizing enterocolitis - a meta-analysis. Eur Rev Med Pharmacol Sci 2016;20:3902-10. [PubMed]
- Rath ME, Smits-Wintjens VE, Lindenburg I, et al. Top-up transfusions in neonates with Rh hemolytic disease in relation to exchange transfusions. Vox Sang 2010;99:65-70. [Crossref] [PubMed]
- al-Alaiyan S, al Omran A. Late hyporegenerative anemia in neonates with rhesus hemolytic disease. J Perinat Med 1999;27:112-5. [Crossref] [PubMed]
- De Boer IP, Zeestraten EC, Lopriore E, et al. Pediatric outcome in Rhesus hemolytic disease treated with and without intrauterine transfusion. Am J Obstet Gynecol 2008;198:54.e1-4. [Crossref] [PubMed]
- DeMoss P, Asfour M, Hersey K. Anti-K1 (Kell) antibody expressed in maternal breastmilk: a case report of a neonate with multiple intrauterine transfusions and postnatal exposure to kell antibody in maternal breastmilk. Case Rep Pediatr 2017;2017:6927813. [Crossref] [PubMed]
- Li M, Blaustein JC. Persistent hemolytic disease of the fetus and newborn (HDFN) associated with passive acquisition of anti-D in maternal breast milk. Transfusion 2017;57:2121-4. [Crossref] [PubMed]
- Leonard A, Hittson Boal L, Pary P, et al. Identification of red blood cell antibodies in maternal breast milk implicated in prolonged hemolytic disease of the fetus and newborn. Transfusion 2019;59:1183-9. [Crossref] [PubMed]
- Miescher S, Spycher MO, Amstutz H, et al. A single recombinant anti-RhD IgG prevents RhD immunization: association of RhD-positive red blood cell clearance rate with polymorphisms in the FcgammaRIIA and FcgammaIIIA genes. Blood 2004;103:4028-35. [Crossref] [PubMed]
- Kumpel BM. Efficacy of RhD monoclonal antibodies in clinical trials as replacement therapy for prophylactic anti-D immunoglobulin: more questions than answers. Vox Sang 2007;93:99-111. [Crossref] [PubMed]
- McMaster conference on prevention of Rh immunization. 28-30 September, 1977. Vox Sang 1979;36:50-64. [Crossref] [PubMed]
- Xie X, Fu Q, Bao Z, et al. Clinical value of different anti-D immunoglobulin strategies for preventing Rh hemolytic disease of the fetus and newborn: A network meta-analysis. PLoS One 2020;15:e0230073. [Crossref] [PubMed]
- Silver RM. RhD immune globulin: over 50 years of remarkable progress! BJOG 2016;123:1347. [Crossref] [PubMed]
- Sørensen K, Baevre MS, Tomter G, et al. The Norwegian experience with nationwide implementation of fetal RHD genotyping and targeted routine antenatal anti-D prophylaxis. Transfus Med 2021;31:314-21. [Crossref] [PubMed]
- Sandler SG, Chen LN, Flegel WA. Serological weak D phenotypes: a review and guidance for interpreting the RhD blood type using the RHD genotype. Br J Haematol 2017;179:10-9. [Crossref] [PubMed]
- Kacker S, Vassallo R, Keller MA, et al. Financial implications of RHD genotyping of pregnant women with a serologic weak D phenotype. Transfusion 2015;55:2095-103. [Crossref] [PubMed]
- Sandler SG, Flegel WA, Westhoff CM, et al. It's time to phase in RHD genotyping for patients with a serologic weak D phenotype. College of American Pathologists Transfusion Medicine Resource Committee Work Group. Transfusion 2015;55:680-9. [Crossref] [PubMed]
- Kim YA, Makar RS. Detection of fetomaternal hemorrhage. Am J Hematol 2012;87:417-23. [Crossref] [PubMed]
- Pelikan DM, Scherjon SA, Mesker WE, et al. Quantification of fetomaternal hemorrhage: a comparative study of the manual and automated microscopic Kleihauer-Betke tests and flow cytometry in clinical samples. Am J Obstet Gynecol 2004;191:551-7. [Crossref] [PubMed]
- Pham HP, Marques MB, Williams LA 3rd. Rhesus Immune Globulin Dosing in the Obesity Epidemic Era. Arch Pathol Lab Med 2015;139:1084. [Crossref] [PubMed]
- Woo EJ, Kaushal M. Rhesus Immunoglobulin Dosage and Administration in Obese Individuals. Arch Pathol Lab Med 2017;141:17. [Crossref] [PubMed]
- O’Brien KL, Kim YA, Haspel RL, et al. Provision of KEL1-negative blood to obstetric patients: a 3-year single-institution retrospective review. Transfusion 2015;55:599-604; quiz 598. [Crossref] [PubMed]
- Oud JA, Evers D, de Haas M, et al. The effect of extended c, E and K matching in females under 45years of age on the incidence of transfusion-induced red blood cell alloimmunisation. Br J Haematol 2021;195:604-11. [Crossref] [PubMed]
- Watson WJ, Wax JR, Miller RC, et al. Prevalence of new maternal alloantibodies after intrauterine transfusion for severe Rhesus disease. Am J Perinatol 2006;23:189-92. [Crossref] [PubMed]
- Schonewille H, Klumper FJ, van de Watering LM, et al. High additional maternal red cell alloimmunization after Rhesus- and K-matched intrauterine intravascular transfusions for hemolytic disease of the fetus. Am J Obstet Gynecol 2007;196:143.e1-6. [Crossref] [PubMed]
- Schonewille H, Prinsen-Zander KJ, Reijnart M, et al. Extended matched intrauterine transfusions reduce maternal Duffy, Kidd, and S antibody formation. Transfusion 2015;55:2912-9; quiz 2911. [Crossref] [PubMed]
- Royal College of Obstetricians and Gynaecologists. Antepartum Haemorrhage (Green-top Guideline No. 63). 2011.
- Deutsch AB, Lynch O, Alio AP, et al. Increased risk of placental abruption in underweight women. Am J Perinatol 2010;27:235-40. [Crossref] [PubMed]
- Pariente G, Wiznitzer A, Sergienko R, et al. Placental abruption: critical analysis of risk factors and perinatal outcomes. J Matern Fetal Neonatal Med 2011;24:698-702. [Crossref] [PubMed]
- Abedzadeh-Kalahroudi M. Prevention of postpartum hemorrhage: our options. Nurs Midwifery Stud 2015;4:e29641. [Crossref] [PubMed]
- Gonzalez-Brown V, Schneider P. Prevention of postpartum hemorrhage. Semin Fetal Neonatal Med 2020;25:101129. [Crossref] [PubMed]
- Organization WH. WHO recommendatioins for the prevention and treatment or postpartum haemorrhage: evidence base. World Health Organization; 2012.
- American College of Obstetricians and Gynecologists. ACOG Practice Bulletin: Clinical Management Guidelines for Obstetrician-Gynecologists Number 76, October 2006: postpartum hemorrhage. Obstet Gynecol 2006;108:1039-47. [PubMed]
- Oyelese Y, Ananth CV. Postpartum hemorrhage: epidemiology, risk factors, and causes. Clin Obstet Gynecol 2010;53:147-56. [Crossref] [PubMed]
- Higgins N, Patel SK, Toledo P. Postpartum hemorrhage revisited: new challenges and solutions. Curr Opin Anaesthesiol 2019;32:278-84. [Crossref] [PubMed]
- Alexander JM, Sarode R, McIntire DD, et al. Whole blood in the management of hypovolemia due to obstetric hemorrhage. Obstet Gynecol 2009;113:1320-6. [Crossref] [PubMed]
- Pitman JP, Wilkinson R, Liu Y, et al. Blood component use in a sub-Saharan African country: results of a 4-year evaluation of diagnoses associated with transfusion orders in Namibia. Transfus Med Rev 2015;29:45-51. [Crossref] [PubMed]
- Borgman MA, Spinella PC, Perkins JG, et al. The ratio of blood products transfused affects mortality in patients receiving massive transfusions at a combat support hospital. J Trauma 2007;63:805-13. [Crossref] [PubMed]
- Holcomb JB, Tilley BC, Baraniuk S, et al. Transfusion of plasma, platelets, and red blood cells in a 1:1:1 vs a 1:1:2 ratio and mortality in patients with severe trauma: the PROPPR randomized clinical trial. JAMA 2015;313:471-82. [Crossref] [PubMed]
- Goucher H, Wong CA, Patel SK, et al. Cell Salvage in Obstetrics. Anesth Analg 2015;121:465-8. [Crossref] [PubMed]
- Khan KS, Moore P, Wilson M, et al. A randomised controlled trial and economic evaluation of intraoperative cell salvage during caesarean section in women at risk of haemorrhage: the SALVO (cell SALVage in Obstetrics) trial. Health Technol Assess 2018;22:1-88. [Crossref] [PubMed]
- O'Brien KL, Shainker SA, Lockhart EL. Transfusion Management of Obstetric Hemorrhage. Transfus Med Rev 2018;32:249-55. [Crossref] [PubMed]
- Committee on practice bulletins-obstetrics. Practice bulletin no. 183: postpartum hemorrhage. Obstet Gynecol 2017;130:e168-e86. [Crossref]
- Bryant-Smith AC, Lethaby A, Farquhar C, et al. Antifibrinolytics for heavy menstrual bleeding. Cochrane Database Syst Rev 2018;4:CD000249. [PubMed]
- Kruithof EK, Tran-Thang C, Gudinchet A, et al. Fibrinolysis in pregnancy: a study of plasminogen activator inhibitors. Blood 1987;69:460-6. [Crossref] [PubMed]
- WOMAN Trial Collaborators. Effect of early tranexamic acid administration on mortality, hysterectomy, and other morbidities in women with post-partum haemorrhage (WOMAN): an international, randomised, double-blind, placebo-controlled trial. Lancet 2017;389:2105-16. [Crossref] [PubMed]
- Shakur H, Beaumont D, Pavord S, et al. Antifibrinolytic drugs for treating primary postpartum haemorrhage. Cochrane Database Syst Rev 2018;2:CD012964. [Crossref] [PubMed]
- WHO Guidelines approved by the Guidelines Review Committee. WHO recommendation on tranexamic acid for the treatment of postpartum haemorrhage. Geneva: World Health Organization, 2017.
- Butwick A, Lyell D, Goodnough L. How do I manage severe postpartum hemorrhage? Transfusion 2020;60:897-907. [Crossref] [PubMed]
- Sudhof LS, Shainker SA, Einerson BD. Tranexamic acid in the routine treatment of postpartum hemorrhage in the United States: a cost-effectiveness analysis. Am J Obstet Gynecol 2019;221:275.e1-275.e12. [Crossref] [PubMed]
- Sentilhes L, Winer N, Azria E, et al. Tranexamic acid for the prevention of blood loss after vaginal delivery. N Engl J Med 2018;379:731-42. [Crossref] [PubMed]
- Sentilhes L, Sénat MV, Le Lous M, et al. Tranexamic acid for the prevention of blood loss after cesarean delivery. N Engl J Med 2021;384:1623-34. [Crossref] [PubMed]
- Villers MS, Jamison MG, De Castro LM, et al. Morbidity associated with sickle cell disease in pregnancy. Am J Obstet Gynecol 2008;199:125.e1-5. [Crossref] [PubMed]
- Chang JN, Magann EF, Novotny SA, et al. Maternal/perinatal outcome in women with sickle cell disease: a comparison of two time periods. South Med J 2018;111:742-5. [Crossref] [PubMed]
- Barfield WD, Barradas DT, Manning SE, et al. Sickle cell disease and pregnancy outcomes: women of African descent. Am J Prev Med 2010;38:S542-9. [Crossref] [PubMed]
- Oteng-Ntim E, Meeks D, Seed PT, et al. Adverse maternal and perinatal outcomes in pregnant women with sickle cell disease: systematic review and meta-analysis. Blood 2015;125:3316-25. [Crossref] [PubMed]
- Alayed N, Kezouh A, Oddy L, et al. Sickle cell disease and pregnancy outcomes: population-based study on 8.8 million births. J Perinat Med 2014;42:487-92. [Crossref] [PubMed]
- Ballas SK, McCarthy WF, Guo N, et al. Exposure to hydroxyurea and pregnancy outcomes in patients with sickle cell anemia. J Natl Med Assoc 2009;101:1046-51. [Crossref] [PubMed]
- Oakley LL, Awogbade M, Brien S, et al. Serial prophylactic exchange blood transfusion in pregnant women with sickle cell disease (TAPS-2): study protocol for a randomised controlled feasibility trial. Trials 2020;21:347. [Crossref] [PubMed]
- Koshy M, Burd L, Wallace D, et al. Prophylactic red-cell transfusions in pregnant patients with sickle cell disease. A randomized cooperative study. N Engl J Med 1988;319:1447-52. [Crossref] [PubMed]
- Chou ST, Alsawas M, Fasano RM, et al. American Society of Hematology 2020 guidelines for sickle cell disease: transfusion support. Blood Adv 2020;4:327-55. [Crossref] [PubMed]
- Smith-Whitley K. Complications in pregnant women with sickle cell disease. Hematology Am Soc Hematol Educ Program 2019;2019:359-66. [Crossref] [PubMed]
- Coleman S, Westhoff CM, Friedman DF, et al. Alloimmunization in patients with sickle cell disease and underrecognition of accompanying delayed hemolytic transfusion reactions. Transfusion 2019;59:2282-91. [Crossref] [PubMed]
- Werner EJ, Broxson EH, Tucker EL, et al. Prevalence of von Willebrand disease in children: a multiethnic study. J Pediatr 1993;123:893-8. [Crossref] [PubMed]
- Rodeghiero F, Castaman G, Dini E. Epidemiological investigation of the prevalence of von Willebrand's disease. Blood 1987;69:454-9. [Crossref] [PubMed]
- Sánchez-Luceros A, Meschengieser SS, Marchese C, et al. Factor VIII and von Willebrand factor changes during normal pregnancy and puerperium. Blood Coagul Fibrinolysis 2003;14:647-51. [Crossref] [PubMed]
- Huq FY, Kulkarni A, Agbim EC, et al. Changes in the levels of factor VIII and von Willebrand factor in the puerperium. Haemophilia 2012;18:241-5. [Crossref] [PubMed]
- Govorov I, Löfgren S, Chaireti R, et al. Postpartum hemorrhage in women with von willebrand disease - a retrospective observational study. PLoS One 2016;11:e0164683. [Crossref] [PubMed]
- Delbrück C, Miesbach W. The Course of von Willebrand Factor and Factor VIII Activity in Patients with von Willebrand Disease during Pregnancy. Acta Haematol 2019;142:71-8. [Crossref] [PubMed]
- Leebeek FWG, Duvekot J, Kruip MJHA. How I manage pregnancy in carriers of hemophilia and patients with von Willebrand disease. Blood 2020;136:2143-50. [Crossref] [PubMed]
- James AH, Jamison MG. Bleeding events and other complications during pregnancy and childbirth in women with von Willebrand disease. J Thromb Haemost 2007;5:1165-9. [Crossref] [PubMed]
- Nichols WL, Hultin MB, James AH, et al. von Willebrand disease (VWD): evidence-based diagnosis and management guidelines, the National Heart, Lung, and Blood Institute (NHLBI) Expert Panel report (USA). Haemophilia 2008;14:171-232. [Crossref] [PubMed]
- Laffan MA, Lester W, O'Donnell JS, et al. The diagnosis and management of von Willebrand disease: a United Kingdom Haemophilia Centre Doctors Organization guideline approved by the British Committee for Standards in Haematology. Br J Haematol 2014;167:453-65. [Crossref] [PubMed]
- Committee on Adolescent Health Care. Committee Opinion No.580: von Willebrand disease in women. Obstet Gynecol 2013;122:1368-73. [Crossref] [PubMed]
- O'Brien SH, Stanek JR, Kaur D, et al. Laboratory monitoring during pregnancy and post-partum hemorrhage in women with von Willebrand disease. J Thromb Haemost 2020;18:604-8. [Crossref] [PubMed]
- Machin N, Ragni MV. Recombinant vs plasma-derived von Willebrand factor to prevent postpartum hemorrhage in von Willebrand disease. Blood Adv 2020;4:3234-8. [Crossref] [PubMed]
- James AH, Konkle BA, Kouides P, et al. Postpartum von Willebrand factor levels in women with and without von Willebrand disease and implications for prophylaxis. Haemophilia 2015;21:81-7. [Crossref] [PubMed]
- Stoof SC, van Steenbergen HW, Zwagemaker A, et al. Primary postpartum haemorrhage in women with von Willebrand disease or carriership of haemophilia despite specialised care: a retrospective survey. Haemophilia 2015;21:505-12. [Crossref] [PubMed]
- Ragni MV, Machin N, James AH, et al. Feasibility of the Von Willebrand disease PREVENT trial. Thromb Res 2017;156:8-13. [Crossref] [PubMed]
- Garagiola I, Mortarino M, Siboni SM, et al. X Chromosome inactivation: a modifier of factor VIII and IX plasma levels and bleeding phenotype in Haemophilia carriers. Eur J Hum Genet 2021;29:241-9. [Crossref] [PubMed]
- Nau A, Gillet B, Guillet B, et al. Bleeding complications during pregnancy and delivery in haemophilia carriers and their neonates in Western France: An observational study. Haemophilia 2020;26:1046-55. [Crossref] [PubMed]
- Paroskie A, Gailani D, DeBaun MR, et al. A cross-sectional study of bleeding phenotype in haemophilia A carriers. Br J Haematol 2015;170:223-8. [Crossref] [PubMed]
- Srivastava A, Brewer AK, Mauser-Bunschoten EP, et al. Guidelines for the management of hemophilia. Haemophilia 2013;19:e1-47. [Crossref] [PubMed]
- Management of Inherited Bleeding Disorders in Pregnancy: Green-top Guideline No. 71 (joint with UKHCDO). BJOG 2017;124:e193-263. [Crossref] [PubMed]
- Kadir RA, Economides DL, Braithwaite J, et al. The obstetric experience of carriers of haemophilia. Br J Obstet Gynaecol 1997;104:803-10. [Crossref] [PubMed]
- Chi C, Lee CA, Shiltagh N, et al. Pregnancy in carriers of haemophilia. Haemophilia 2008;14:56-64. [PubMed]
- Thornton P, Douglas J. Coagulation in pregnancy. Best Pract Res Clin Obstet Gynaecol 2010;24:339-52. [Crossref] [PubMed]
- Casini A, Blondon M, Lebreton A, et al. Natural history of patients with congenital dysfibrinogenemia. Blood 2015;125:553-61. [Crossref] [PubMed]
- Bouttefroy S, Meunier S, Milien V, et al. Congenital factor XIII deficiency: comprehensive overview of the FranceCoag cohort. Br J Haematol 2020;188:317-20. [Crossref] [PubMed]
Cite this article as: Linder GE, Ipe TS. Pregnancy and postpartum transfusion. Ann Blood 2022;7:12.