Atypical hemolytic uremic syndrome: genetically-based insights into pathogenesis through an analysis of the complement regulator CD46
Introduction
Hemolytic uremic syndrome (HUS) is a thrombotic microangiopathy (TMA) characterized by a triad of microangiopathic hemolytic anemia (MAHA), thrombocytopenia and acute renal failure (ARF). HUS is classified into two major types—‘typical’ or Shiga toxin-producing Escherichia coli infection and ‘atypical’ HUS (aHUS). In comparison to bacterial-toxin-related HUS, aHUS has a worse prognosis. Herein we will focus on recent developments in our understanding of the role of the complement system, particularly one of its key regulators, CD46, in the pathogenesis of aHUS. The complement system was originally implicated in aHUS based on the observation that a subset of aHUS patients (especially in familial situations) had low C3, implicating complement consumption in its pathogenesis (1,2).
In the past twenty years, thanks largely to modern genetics, an impressive story relative to the pathogenesis of aHUS has emerged in that over 50% of aHUS cases are due to excessive alternative pathway (AP) activation (see “Complement activation” section below) (3,4). We and others term this clinical syndrome as “Complement mediated aHUS (C-aHUS)”. Cases can be divided into loss-of-function (LOF) mutations of complement regulatory proteins (the large majority); namely, CD46 (MCP), CFH or CFI, and gain-of-function (GOF) mutations in AP activating proteins C3 or factor B (CFB). To date, more than 500 variants associated with aHUS have been identified in these five complement proteins with approximately 47–60% being variants in CFH or MCP. Recent literature reviews indicate that complement protein mutations that contribute to the pathology of aHUS are as follows: CFH 40–45%, CD46 10–15%, CFI 5–15%, C3 5–15%, and CFB 1–5% (2,3). Up to 20% of aHUS patients have more than one complement-related gene mutation. Autoantibodies against factor H or factor I are also involved in a few cases (5), but no autoantibodies to CD46 have been identified (6). Throughout our review, we will note genes as CD46, CFH or CFI, but proteins as CD46, FH or FI.
Mutations in coagulation system proteins, especially thrombomodulin, have also been identified in patients with aHUS (6). As a cell surface protein, thrombomodulin is involved in anticoagulation and modulating complement activation (6). However, this interesting association will not be addressed here as our focus will be on CD46.
Complement activation
The complement system is a player in the innate immune system that developed early in evolution (7). When foreign pathogenic microorganisms invade, complement can be rapidly activated. In two to five mins, it can deposit up to several million complement-activation fragments on a pathogen's membrane. Two common triggers for this mechanism are lectins and antibodies. This ‘opsonization’ initiates immune adherence mechanisms followed by phagocytosis or lysis [for a review of the complement system see studies by Atkinson et al. and Pangburn (7,8)]. The magnitude and rapidity of activation are critical features of complement function in host defense. Further, the complement system is also a bridge between innate and adaptive immune responses as C3-activated products are natural adjuvants to identify foreign antigens and to lower the threshold of B cell activation (7). Thus, C3-deficient mice and humans are impaired in forming germinal centers and producing antibodies following antigen challenge (9).
The activation of complement classical (CP) and lection pathways (LP) are mediated mainly by antibody and lectins, respectively. The latter interact with carbohydrate components of microbes. The AP may be activated by spontaneous hydrolysis (tickover/turnover) of C3’s thioester bond to form C3 (H2O). It is also a powerful amplification loop that enhances the initiative activation by CP and/or LP. Employing C5 activation as a measurement for total complement activation in several well studied examples, the AP contributed up to 80% of its generation (7). This point further illustrates the importance of tight regulation of AP.
Complement is a surveillance system. It is continuously on alert for invasion by pathogenic microorganisms through a continuous generation of a fluid phase AP C3 convertase C3(H2O)Bb (Figure 1). Via hydrolysis of C3 (1% to 2%/h), C3(H2O) binds plasma factor B (FB) to form AP convertase precursor C3 convertase C3(H2O)B. Plasma serine protease factor D (FD) then cleaves FB into Ba (released) and Bb to form a fluid phase AP convertase C3(H2O)Bb. This enzyme then cleaves C3 to C3b which in turn covalently attaches to a nearby target to trigger local complement activation (the amplification loop).
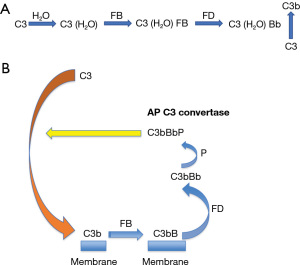
Complement regulation—cofactor activity (CA) and decay accelerating activity (DAA)
On account of its powerful and rapid response and to ensure that its activation does not lead to excessive collateral damage to host tissues, the regulators of complement activation (RCA) employ two overall inhibitory strategies: one of these is CA in which regulatory proteins engage the plasma serine protease, FI, to cleave and thereby inactivate C3b and C4b deposited on host cells (Figure 2). The three main cofactor proteins responsible for regulating C4b and/or C3b in concert with FI are CD46, FH and C3b/C4b receptor-complement receptor 1 (CR1; CD35). CD46 and CR1 are membrane proteins that protect the cell from complement-mediated damage. CD46 cleaves C3b and C4b deposited on the same cell membrane on which it is expressed (7). CD46 is expressed on most cell types with the notable exception of red blood cells (RBCs) while CR1 has limited expression, primarily peripheral blood cells (including RBCs) and tissue macrophages and podocytes. CR1 also serves as the immune adherence receptor on RBCs. Thus, “like a taxi”, the CR1-RBC binds immune complexes and shuttles them to the phagocytic system where the immune complex is transferred to macrophages in the liver and spleen. FH is a fluid-phase protein but also binds to membranes. Thus, FH is a powerful inhibitor of complement activation working in both the fluid and solid membrane phases (10). FH binds to host cell membranes and tissue/foreign debris through its glycosaminoglycans and sialic acid binding domains as well as by engaging C3b, iC3b and C3d. Interestingly, we want to point out that only variants in complement regulatory proteins with CA are involved in the pathogenesis of aHUS.
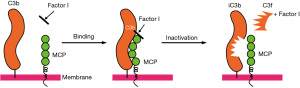
A second mechanism to control complement activation is DAA. As noted above and in contrast to regulators mediating CA, no variant or mutation in decay accelerating factor (CD55/DAF) has been identified in the aHUS patients. Why does CD55 deficiency not have an impact on aHUS? The biological function of CD55 is to prevent the formation of and to accelerate the decay of C3 and C5 convertases. Although CD55 decays these convertases, the “dissociated” C3b and C4b can reform another convertase. Thus, the binding of the C3b and C4b on the cell membrane is covalent and permanent, requiring immediate and definitive inactivation by CA. Regulating the “effector molecules” such as C3b and C4b is a more permanent solution vs. decaying a convertase.
aHUS
CFH—historical aspects
As early as 1981, aHUS was identified in a family with complete FH deficiency (11). However, a major observation in 1998 that “broke open the field” was by the Goodship team in Newcastle in the United Kingdom. They identified heterogeneous mutations in FH that were associated with aHUS (4). They also localized aHUS pathogenesis to a genetic locus on the long arm of chromosome 1q32 (12). This locus contains multiple complement regulatory proteins including FH, CD46, CR1 and CD55. The RCA gene cluster at 1q32 also shares functional similarities in that they consist largely of multiple homologous short consensus repeats [SCR, complement control protein (CCP) or sushi domains] as their primary amino acid structure. The approximately 60 amino acid repeats are the sites for complement regulation. While CD46 only has CA in regulation of C3b/C4b, FH has both DAA for C3 and C5 convertases and CA for C3b.
CD46—historical aspects
As a membrane protein, CD46 plays an “intrinsic role” by inactivating C3b and C4b deposited on the same cell surface on which it is expressed (13,14). The role of CD46 in aHUS was first established in 2003 with the identification of a six-nucleotide deletion in a heterozygous mutation resulting in the loss of D271/S272 (previously numbered as D237/S238) (Figure 3) (16). Further studies determined that this mutation caused CD46 protein to accumulate in the cytoplasm leading to a decrease (haploinsufficiency) in its cell membrane expression (15,17,18).

One aim of our review is to provide on a prior comprehensive review (American, Italian, and French cohorts) on the initial mutations in membrane cofactor protein (MCP/CD46) in aHUS which is now commonly called complement-mediated aHUS (C-aHUS) (19). In that review published in 2003, the authors characterized the clinical and pathologic findings in first 23 rare genetic variants in 30 families (19).
Several important findings were summarized in that review (19). Firstly, among two-thirds of the variants, there were approximately 50% with a significant reduction of the protein expression. Moreover, over 90% of the mutations were in the four repeats where the binding sites for C3b/C4b are located. To gain a comprehensive understanding of these variants, patient samples were evaluated by flow cytometry, Western blotting and enzyme-linked immunosorbent assay (ELISA). In addition, the binding of recombinant variants with C3b/C4b binding and their subsequent CA were assessed. Also, overall analysis revealed that patients with the CD46 variant had a better clinical outcome than those with a rare variant of CFH or CFI.
These data have been validated with no major differences in the CD46 cohort which now features nearly 80 variants (Figure 3). Consequently, we will focus our discussion on new therapies and renal transplantation. A unique feature, of course, relative to a CD46 cohort is that kidney transplantation delivers an organ with normal CD46 expression. Further more rapid screening is now available for identifying the mutation and >90% of patients respond to anti-C5 monoclonal antibody therapy (15,16).
CD46—structure
CD46 is a transmembrane protein that is expressed on almost all cells with the notable exception of erythrocytes [reviewed in study by Richards et al. (19)]. CD46 has four extracellular CCP repeats (Figure 3). This is followed by an alternatively spliced region enriched in serines, threonines and prolines (STP) that is a site for O-glycosylation. Next is a small segment of unknown function followed by an alternatively spliced cytoplasmic tail (CYT-1 or CYT-2) that each possess a distinct signaling motifs (19).
Function
CD46 interacts with the plasma protease FI to cleave C3b into the inactive fragment iC3b (Figure 2). The importance of this activity lies in FI’s ability to permanently inactivate C3b deposited on the cell membrane so that the enzyme (a C3 convertase) cannot be formed and thereby induce subsequent complement interactions to cleave C3 and C5. Thus, CD46 serves to efficiently regulate the amplification loop (AP C3 convertase) to prevent complement-mediated self-tissue damage.
Approximately 80 rare CD46 variants have been associated with aHUS: 4 in the SP, 12 in CCP1, 15 in CCP2, 16 in CCP3, and 16 in CCP4, while in other regions there are 2 in the promoter, 4 in the signal peptide, 1 in the STP, 5 in the transmembrane domain, and 2 in the CYT-1 (see Figure 3) (16). Most of the CD46 mutations are heterozygous and lead to either a decrease in cell membrane expression (approximately 75%) or have normal expression but a loss of functional activity (about 25%) (15,16,19).
Receptor for pathogens
Due to its wide range of cell expression and protective functional activity, CD46 also serves as the receptor or adherence factor for at least 11 pathogens (five viruses and six bacteria) (15). Because of this, CD46 has been called a “pathogen magnet” (15). CD46 is a receptor for measles virus (MV) and multiple strains of adenoviruses (AdV). Species of AdV and MV that target CD46 are being developed for a wide range of therapeutic interventions (15).
C3 levels and CD46 deficiency
Of interest, the majority of aHUS patients with CD46 mutations (63–89%) have normal C3 levels (15). Because mouse CD46 is only expressed in the eye and on spermatozoa (20), mouse membrane regulator Crry, a functional counterpart of human CD46, has a role in maintaining the homeostasis of complement serum levels. Crry null mice have significantly lower levels of C3 (21). However, patients with complete CD46 deficiency due to R25X and Y214X mutations had C3 levels in the normal range, suggesting that CD46 has a minimal role in controlling the complement tick-over process in the plasma in man (22,23).
Clinical presentation
Patients with CD46 mutations generally show milder disease than those with CFH or CFI mutations and are less prone to developing end-stage renal disease (ESRD) (15). Due to CD46 being expressed normally in the renal tissues (i.e., epithelial, endothelial and mesangial cells), patients with CD46 mutations who underwent renal transplantation experienced a low relapse rate (<20%). In contrast, patients with mutations in CFH or CFI genes had an approximately 80% recurrence rate following renal transplantation (22,24).
A specific MCP single nucleotide polymorphism block in the promoter region, termed the MCPggaac risk haplotype, has been associated with lower transcriptional activity and also been linked to aHUS, but only in the setting of a causative variant in another complement regulator or AP component (25). Additionally, kidney transplant recipients bearing the risk haplotype with de novo TMA were found to be at an increased risk of graft loss (26).
Complete deficiency
Regarding the complete deficiency of CD46, in addition to aHUS, three of the eight patients identified have common variable immunodeficiency (27). The other five people though did have lower levels of IgG1 and further studies found that the T cells of CD46-deficient patients were hindered in stimulating B cells to produce antibodies (27).
Other complement components and aHUS
FH
Although this review focuses on CD46 in aHUS, other rare variants of complement proteins are also implicated as mentioned earlier. Thus, FH is a large (150 kDa) and abundant plasma complement regulatory protein composed of 20 SCRs (22). The CA of FH is located in SCRs 1–4 of the amino-terminus, whereas its SCR 6–7 and SCR 19–20 have a recognition function that allows FH to bind to oligosaccharides on membranes. This allows FH to have protective roles both in the fluid phase and on cells. It provides an explanation as to why aHUS patients with CFH mutations have a worse clinical outcome (23). As with CD46, most all the CFH mutations are heterozygous.
FI
FI is a plasma serine protease. Its synthesis by the liver and endothelial cells can be modulated by proinflammatory cytokines such as interleukin-6 (IL-6) and interferon gamma (IFN-γ), demonstrating that FI is also an acute phase protein (7,22). Its important function is to work with a membrane and fluid-phase regulators (FH, CD46, CR1 and C4BP) to cleave C3b and/or C4b into inactive components relative to further complement activation (Figure 2). It is impressive to note that, as per CFH and CD46, CFI mutations associated with aHUS are heterozygous (23,28-31). The clinical prognosis of CFI variants on aHUS is similar to CFH variants. One study showed that long-term maintenance of renal function for CD46, CFH and CFI mutations were 86, 23% and 33%, respectively (22).
FB and GOF
FB binds to C3b and then FD cleaves it to form the AP C3 convertase C3bBb (Figure 1). A GOF mutation of CFB leads to a more powerful convertase, being more difficult to inhibit by FH. From structural biology considerations, mutations that lead to a GOF occur within the binding site of FB to C3b, or locations where electronic repulsion occurs between FB and FH. Thus, CFB mutations with a GOF facilitate C3 and C5 convertase formation (32-34).
C3 and gain of function
C3 is one of the most abundant proteins in blood. It is the “convergent” protein of the three major pathways of complement activation. C3 is an “effector factor” because its degradation products have various biological functions that range from chemotaxis (C3a) to opsonization (C3b). Also, its covalently bound degradation products (iC3b, C3dg, C3d) interact with complement receptors on macrophages and lymphocytes (7). Mutations in C3 lead to an increase in the binding of FB to form a hyperactive C3 convertase secondary to a decrease in C3b cleavage by regulators. These patients with C3 GOF enhance complement activation that results in rapidly developing ESRD and a high frequency of cardiac and neurological manifestations (25,35-37).
Combined mutations
Combined mutations in complement proteins generally exhibit more severe disease than single gene mutations and also pose more challenges for treatment (25,36,37). Combined mutations in the cohorts in Italy and France were 3.4% and 4.2%, respectively (22,38-40). In Italian cohorts, combined mutations in CD46/CFI accounted for up to 25% of patients (22,38-40).
Complement regulation and kidney
In aHUS, the kidney is the target organ of complement-mediated inflammatory damage. About 50–70% of patients will develop ESRD if they do not receive timely treatment. Although the exact mechanism as to why glomerular endothelium is the main target in aHUS is not entirely clear, it is speculated that the fenestrations in the endothelium make it more susceptible to complement dysregulation since the underlying glomerular basement membrane lacks complement regulators (2,41,42).
CD46, CD59 and CD55 are expressed in glomerular cells (43). CD55 is though expressed in the juxtaglomerular apparatus (JGA), while CR1 expression is limited to podocytes (44-46). By immunohistochemistry, CD46 is widely expressed in various cell types in the kidney, and it has also been shown to have CA in glomerular epithelial cells and mesangial cells (43-45). Considering that CR1 is localized to podocytes, a type of epithelial cell, CD46 is the only widely expressed membrane protein in kidney capable of performing CA. However, specific expression of its different isoforms could be related to disease mechanisms (46). These expression patterns should have pathological significance because the main site of tissue damage in aHUS is the glomerulus. FH deficiency caused by variants may significantly impact the kidney since it not only is a plasma protein but it can also attach to cell membranes to exercise its role as a membrane regulatory protein with CA.
Interestingly, in contrast to the importance of CA, several mutations identified in CD55 that reduces DAA did not contribute to the pathogenesis of aHUS. Also, no mutations in aHUS have been found in membrane regulators of complement activation CR1 and CD59 (24,42).
Summary of complement associations
The LOF in a regulator or a GOF in an activator upsets the natural homeostasis of a finely tuned and regulated complement system. Such dysfunction particularly leads to the loss of protection of endothelial cells and subsequent overactivation of complement in various organs, primarily in the kidneys (3). However, penetrance of these mutations often requires a secondary trigger from other genetic perturbations, initiating diseases (such as infection), pregnancy, or environmental factors (such as a medication) that together with a rare complement variant will induce aHUS (47). The heterozygous state of the mutation unequivocally establishes how key it is to have “normal” levels of these regulators. Haploinsufficiency doesn’t get the job done! Thus, for patients suspected of having aHUS, genetic analyses of complement components are highly recommended to establish diagnosis and to inform treatment options. Of special note, renal transplantation for patients with CD46 mutations provides a higher probability of organ survival than those with mutations in FH or FI, since the transplanted kidney carries a normal CD46 (25,48,49).
Pathogenesis of aHUS-lessons learned
Reduced control of complement activation (specifically CA) on the cell membrane is the essential pathogenetic mechanism of aHUS. Mutations of membrane regulator CD46 or serum protease CFI contribute to inadequate regulation of complement activation on the cell membrane (24). Also, many of the CFH mutations associated with aHUS occur in its C-terminal region (CCPs 16–20) where FH binds to a cell membrane through glycosaminoglycan (GAG). The phenotype of CFH C-terminal null mutant mice was similar to that of the aHUS while the cross of this mutant mouse into a C5 deficient background led to suppression of disease production (24). Finally, most autoantibodies against FH in aHUS patients predominantly recognize the C-terminus of FH (24). These observations indicate that the lack of effective catabolism of C3b on endothelial cells plays a key role in the pathogenesis of aHUS (Figure 4).
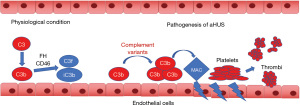
Treatment: anti-C5 monoclonal antibody
Eculizumab, a monoclonal antibody against C5, for aHUS treatment has revolutionized the therapy of aHUS (50-52). Eculizumab binds to C5 and prevents its cleavage to C5a and C5b, thereby preventing formation of the membrane attack complex (MAC), C5b-9, and the liberation of C5a. Substantial evidence points to C5b-C9 causing most of the damage in aHUS. Because it acts downstream of C3, eculizumab preserves the autoimmune protective and immune-enhancing proinflammatory functions of the opsonin C3b and the anaphylatoxin C3a. Treatment with eculizumab should be considered in all patients with aHUS due to a known pathogenic mutation in a complement protein.
Before the approval of eculizumab, there was a recurrence risk of >50% with graft loss occurring in 80% to 90% of aHUS cases. The outcome though varies depending on the complement abnormality. Risk of recurrence is high except in patients carrying CD46 variant. Patients with recurrent aHUS usually present within one year after transplantation and often within days to weeks due to the combined effect of multiple triggers for endothelial injury. Potential triggers at the time of transplantation include ischemia-reperfusion injury, immunosuppressive drugs [calcineurin and mammalian target of rapamycin (mTOR) inhibitors], antibody-mediated rejection (antigen-antibody complexes can activate the complement system), and infections (cytomegalovirus, BK virus, upper respiratory, gastroenteritis) (28).
Treatment: transplantation
Patients with aHUS who progress to end stage kidney disease are candidates for transplantation. Renal transplantation had been contraindicated because of the high risk of disease recurrence leading to early allograft loss especially in patients with heterozygous rare variants in CFH or CFI (28-31). However, over the past decade, advances in our understanding of the pathophysiology of aHUS and availability of highly efficacious anticomplement drugs have permitted successful kidney transplantation in these patients. Therefore, all patients with aHUS should undergo an evaluation to screen for complement system abnormalities (28).
The use of anti-C5 antibodies as a prophylactic or post-transplant medication increases the survival time of transplanted kidneys (28). At present, for almost all transplant patients of aHUS with a complement mutation, the use of prophylactic mAb to C5 is clinically recommended, although there is a lack of consensus on the duration of time of treatment (28). As would be anticipated, patients with CD46 mutations can usually discontinue an anti-C5 therapy a few weeks after transplantation.
In general, the prognosis in transplantation in patients with fluid-phase complement proteins (FH, FI or C3) variants is poor because the mutant proteins in the recipient remain in plasma after the transplantation of healthy kidneys. In contrast, patients with CD46 deficiency have a better outcome when transplanted with a normal kidney. Prior to using anti-C5 mAb, the recurrence rate of aHUS for transplanted kidney recipients with CFH, CFI, C3 and CD46 variants was 75–90%, 45–80%, 50–60%, and <20% respectively (26). To prevent ischemia-reperfusion after transplantation, living donor organs are better than those from deceased donors. However, living related donors are relatively contraindicated in aHUS (unless genetic evaluations are carried out in family members) even though ischemia reperfusion is less. Before the usage of anti-C5 monoclonal antibody in renal transplantation, over 50% of patients with CFH, CFI, or C3 variants receiving a transplanted healthy kidney developed ESRD within a year, and up to 75% developed ESRD or died after three to five years (47). Again, because the donor kidney in the case of CD46 mutation has normal CD46 expression, the donor kidney is largely protected. These results further point to the importance of the local environment once a donor’s is transplanted.
Gene therapy of aHUS
Although no detailed gene therapy has been attempted to date, genetic correction of the deficiency of those complement regulatory proteins holds promise for the treatment of aHUS (3).
Conclusions
We would like to point out three special aspects of the complement association with variants in CD46 and aHUS: one is the importance of CA to control complement activation, especially in the kidney; the second is that almost all of these variants lead to haploinsufficiency; the third is the efficacy of blocking engagement of C5 in the treatment (53).
Acknowledgments
Funding: This work was supported by NIH (Nos. 5 R35 GM136352-02 and 5 RO1 EY028602).
Footnote
Provenance and Peer Review: This article was commissioned by the Guest Editor (X. Long Zheng) for the series “Thrombotic Thrombocytopenic Purpura” published in Annals of Blood. The article has undergone external peer review.
Peer Review File: Available at https://aob.amegroups.com/article/view/10.21037/aob-22-40/prf
Conflicts of Interest: All authors have completed the ICMJE uniform disclosure form (available at https://aob.amegroups.com/article/view/10.21037/aob-22-40/coif). The series “Thrombotic Thrombocytopenic Purpura” was commissioned by the editorial office without any funding or sponsorship. AJ has royalty from UptoDate and received consulting fees from Chinook Therapeutics. AJ serves as Scientific Advisory Board for Alexion AstraZeneca Rare Disease and Novartis Pharmaceuticals. JPA has received the support from 5 R35 GM136352 – NIH Grant – PI, 5 R01 EY028602 – NIH Grant – Co-PI Subaward OSP2019151 and 5 R21 AR076534-02; and received consulting fees from Avidity Partners, Janssen Research & Development, LLC, 4D Molecular Therapeutics, Inc., BioMarin Pharmaceutical Inc., HiBIO, Inc., Autobahn Therapeutic, Inc., Celldex Therapeutics, formerly Avant Immunotherapeutics, Inc., Kypha, Inc., Achillion Pharmaceuticals, Inc., Annexon Biosciences, Inc., Alexion Pharmaceuticals, Inc., Achillion Pharmaceuticals, Inc., Broadwing Bio, Takeda Pharmaceuticals, and Merck KGaA from Atkinson Lab; and Arrowhead Pharmaceuticals, Inc. and Q32 Bio from Dr. John Atkinson. JPA participates in the Advisory Board of Kypha, Inc., Gemini Therapeutics, Compliment Corporation and Q32 Bio and their payments were made to both Atkinson lab and Dr. John Atkinson. JPA has stocks include Gemini Therapeutics, Inc. – SAB, ypha, Inc. – SAB, Compliment Corporation – SAB, and Q32 Bio – SAB. The authors have no other conflicts of interest to declare.
Ethical Statement: The authors are accountable for all aspects of the work in ensuring that questions related to the accuracy or integrity of any part of the work are appropriately investigated and resolved.
Open Access Statement: This is an Open Access article distributed in accordance with the Creative Commons Attribution-NonCommercial-NoDerivs 4.0 International License (CC BY-NC-ND 4.0), which permits the non-commercial replication and distribution of the article with the strict proviso that no changes or edits are made and the original work is properly cited (including links to both the formal publication through the relevant DOI and the license). See: https://creativecommons.org/licenses/by-nc-nd/4.0/.
References
- Noris M, Remuzzi G. Are HUS and TTP genetically determined? Kidney Int 1998;53:1085-6. [Crossref] [PubMed]
- Noris M, Remuzzi G. Atypical hemolytic-uremic syndrome. N Engl J Med 2009;361:1676-87. [Crossref] [PubMed]
- Fakhouri F, Frémeaux-Bacchi V. Thrombotic microangiopathy in aHUS and beyond: clinical clues from complement genetics. Nat Rev Nephrol 2021;17:543-53. [Crossref] [PubMed]
- Warwicker P, Goodship TH, Donne RL, et al. Genetic studies into inherited and sporadic hemolytic uremic syndrome. Kidney Int 1998;53:836-44. [Crossref] [PubMed]
- Valoti E, Alberti M, Iatropoulos P, et al. Rare Functional Variants in Complement Genes and Anti-FH Autoantibodies-Associated aHUS. Front Immunol 2019;10:853. [Crossref] [PubMed]
- Delvaeye M, Noris M, De Vriese A, et al. Thrombomodulin mutations in atypical hemolytic-uremic syndrome. N Engl J Med 2009;361:345-57. [Crossref] [PubMed]
- Atkinson J, Du Clos T, Mold C, et al. The human complement system: basic concepts and clinical relevance. In: Rich RR. editor. Clinical Immunology: Principles and Practice. 5th ed: Elsevier; 2019:299-317.
- Pangburn MK. Initiation of the alternative pathway of complement and the history of "tickover". Immunol Rev 2023;313:64-70. [Crossref] [PubMed]
- Da Costa XJ, Brockman MA, Alicot E, et al. Humoral response to herpes simplex virus is complement-dependent. Proc Natl Acad Sci U S A 1999;96:12708-12. [Crossref] [PubMed]
- Wong EKS, Hallam TM, Brocklebank V, et al. Functional Characterization of Rare Genetic Variants in the N-Terminus of Complement Factor H in aHUS, C3G, and AMD. Front Immunol 2020;11:602284. [Crossref] [PubMed]
- Thompson RA, Winterborn MH. Hypocomplementaemia due to a genetic deficiency of beta 1H globulin. Clin Exp Immunol 1981;46:110-9. [PubMed]
- Lublin DM, Liszewski MK, Post TW, et al. Molecular cloning and chromosomal localization of human membrane cofactor protein (MCP). Evidence for inclusion in the multigene family of complement-regulatory proteins. J Exp Med 1988;168:181-94. [Crossref] [PubMed]
- Barilla-LaBarca ML, Liszewski MK, Lambris JD, et al. Role of membrane cofactor protein (CD46) in regulation of C4b and C3b deposited on cells. J Immunol 2002;168:6298-304. [Crossref] [PubMed]
- Oglesby TJ, Allen CJ, Liszewski MK, et al. Membrane cofactor protein (CD46) protects cells from complement-mediated attack by an intrinsic mechanism. J Exp Med 1992;175:1547-51. [Crossref] [PubMed]
- Liszewski MK, Atkinson JP. Membrane cofactor protein (MCP; CD46): deficiency states and pathogen connections. Curr Opin Immunol 2021;72:126-34. [Crossref] [PubMed]
- Liszewski MK, Atkinson JP. Complement regulator CD46: genetic variants and disease associations. Hum Genomics 2015;9:7. [Crossref] [PubMed]
- Richards A, Kemp EJ, Liszewski MK, et al. Mutations in human complement regulator, membrane cofactor protein (CD46), predispose to development of familial hemolytic uremic syndrome. Proc Natl Acad Sci U S A 2003;100:12966-71. [Crossref] [PubMed]
- Goodship TH, Liszewski MK, Kemp EJ, et al. Mutations in CD46, a complement regulatory protein, predispose to atypical HUS. Trends Mol Med 2004;10:226-31. [Crossref] [PubMed]
- Richards A, Kathryn Liszewski M, Kavanagh D, et al. Implications of the initial mutations in membrane cofactor protein (MCP; CD46) leading to atypical hemolytic uremic syndrome. Mol Immunol 2007;44:111-22. [Crossref] [PubMed]
- Lyzogubov V, Wu X, Jha P, et al. Complement regulatory protein CD46 protects against choroidal neovascularization in mice. Am J Pathol 2014;184:2537-48. [Crossref] [PubMed]
- Wu X, Spitzer D, Mao D, et al. Membrane protein Crry maintains homeostasis of the complement system. J Immunol 2008;181:2732-40. [Crossref] [PubMed]
- Caprioli J, Noris M, Brioschi S, et al. Genetics of HUS: the impact of MCP, CFH, and IF mutations on clinical presentation, response to treatment, and outcome. Blood 2006;108:1267-79. [Crossref] [PubMed]
- Pickering MC, de Jorge EG, Martinez-Barricarte R, et al. Spontaneous hemolytic uremic syndrome triggered by complement factor H lacking surface recognition domains. J Exp Med 2007;204:1249-56. [Crossref] [PubMed]
- Kavanagh D, Goodship TH. Atypical hemolytic uremic syndrome, genetic basis, and clinical manifestations. Hematology Am Soc Hematol Educ Program 2011;2011:15-20. [Crossref] [PubMed]
- Lumbreras J, Subias M, Espinosa N, et al. The Relevance of the MCP Risk Polymorphism to the Outcome of aHUS Associated With C3 Mutations. A Case Report. Front Immunol 2020;11:1348. [Crossref] [PubMed]
- Petr V, Csuka D, Hruba P, et al. MCPggaac haplotype is associated with poor graft survival in kidney transplant recipients with de novo thrombotic microangiopathy. Front Immunol 2022;13:985766. [Crossref] [PubMed]
- Fuchs A, Atkinson JP, Fremeaux-Bacchi V, et al. CD46-induced human Treg enhance B-cell responses. Eur J Immunol 2009;39:3097-109. [Crossref] [PubMed]
- Java A. Peri- and Post-operative Evaluation and Management of Atypical Hemolytic Uremic Syndrome (aHUS) in Kidney Transplantation. Adv Chronic Kidney Dis 2020;27:128-37. [Crossref] [PubMed]
- Java A, Baciu P, Widjajahakim R, et al. Functional Analysis of Rare Genetic Variants in Complement Factor I (CFI) using a Serum-Based Assay in Advanced Age-related Macular Degeneration. Transl Vis Sci Technol 2020;9:37. [Crossref] [PubMed]
- Java A, Pozzi N, Love-Gregory LD, et al. A Multimodality Approach to Assessing Factor I Genetic Variants in Atypical Hemolytic Uremic Syndrome. Kidney Int Rep 2019;4:1007-17. [Crossref] [PubMed]
- Java A, Ren Z, Hu Z, et al. A comprehensive analysis of Factor I variants in atypical hemolytic uremic syndrome (aHUS). Mol Immunol 2018;102:166-7. [Crossref]
- Goicoechea de Jorge E, Harris CL, Esparza-Gordillo J, et al. Gain-of-function mutations in complement factor B are associated with atypical hemolytic uremic syndrome. Proc Natl Acad Sci U S A 2007;104:240-5. [Crossref] [PubMed]
- Marinozzi MC, Vergoz L, Rybkine T, et al. Complement factor B mutations in atypical hemolytic uremic syndrome-disease-relevant or benign? J Am Soc Nephrol 2014;25:2053-65. [Crossref] [PubMed]
- Zhang Y, Kremsdorf RA, Sperati CJ, et al. Mutation of complement factor B causing massive fluid-phase dysregulation of the alternative complement pathway can result in atypical hemolytic uremic syndrome. Kidney Int 2020;98:1265-74. [Crossref] [PubMed]
- Roumenina LT, Jablonski M, Hue C, et al. Hyperfunctional C3 convertase leads to complement deposition on endothelial cells and contributes to atypical hemolytic uremic syndrome. Blood 2009;114:2837-45. [Crossref] [PubMed]
- Martínez-Barricarte R, Heurich M, Valdes-Cañedo F, et al. Human C3 mutation reveals a mechanism of dense deposit disease pathogenesis and provides insights into complement activation and regulation. J Clin Invest 2010;120:3702-12. [Crossref] [PubMed]
- Roumenina LT, Frimat M, Miller EC, et al. A prevalent C3 mutation in aHUS patients causes a direct C3 convertase gain of function. Blood 2012;119:4182-91. [Crossref] [PubMed]
- Bresin E, Rurali E, Caprioli J, et al. Combined complement gene mutations in atypical hemolytic uremic syndrome influence clinical phenotype. J Am Soc Nephrol 2013;24:475-86. [Crossref] [PubMed]
- Furmańczyk-Zawiska A, Kubiak-Dydo A, Użarowska-Gąska E, et al. Compound Haplotype Variants in CFH and CD46 Genes Determine Clinical Outcome of Atypical Hemolytic Uremic Syndrome (aHUS)-A Series of Cases from a Single Family. J Pers Med 2021;11:304. [Crossref] [PubMed]
- Sellier-Leclerc AL, Fremeaux-Bacchi V, Dragon-Durey MA, et al. Differential impact of complement mutations on clinical characteristics in atypical hemolytic uremic syndrome. J Am Soc Nephrol 2007;18:2392-400. [Crossref] [PubMed]
- Blasco M, Guillén-Olmos E, Diaz-Ricart M, et al. Complement Mediated Endothelial Damage in Thrombotic Microangiopathies. Front Med (Lausanne) 2022;9:811504. [Crossref] [PubMed]
- Habas E, Raynani A, Habas A, et al. Hemolytic uremic syndrome: an updated review. Yemen Journal of Medicine 2022;1:6-13. [Crossref]
- Cosio FG, Sedmak DD, Mahan JD, et al. Localization of decay accelerating factor in normal and diseased kidneys. Kidney Int 1989;36:100-7. [Crossref] [PubMed]
- Yoshida Y, Nishi H. The role of the complement system in kidney glomerular capillary thrombosis. Front Immunol 2022;13:981375. [Crossref] [PubMed]
- Nangaku M. Complement regulatory proteins in glomerular diseases. Kidney Int 1998;54:1419-28. [Crossref] [PubMed]
- Thurman JM, Renner B. Dynamic control of the complement system by modulated expression of regulatory proteins. Lab Invest 2011;91:4-11. [Crossref] [PubMed]
- Fakhouri F, Scully M, Ardissino G, et al. Pregnancy-triggered atypical hemolytic uremic syndrome (aHUS): a Global aHUS Registry analysis. J Nephrol 2021;34:1581-90. [Crossref] [PubMed]
- Duineveld C, Verhave JC, Berger SP, et al. Living Donor Kidney Transplantation in Atypical Hemolytic Uremic Syndrome: A Case Series. Am J Kidney Dis 2017;70:770-7. [Crossref] [PubMed]
- Levi C, Frémeaux-Bacchi V, Zuber J, et al. Midterm Outcomes of 12 Renal Transplant Recipients Treated With Eculizumab to Prevent Atypical Hemolytic Syndrome Recurrence. Transplantation 2017;101:2924-30. [Crossref] [PubMed]
- Hillmen P, Young NS, Schubert J, et al. The complement inhibitor eculizumab in paroxysmal nocturnal hemoglobinuria. N Engl J Med 2006;355:1233-43. [Crossref] [PubMed]
- Legendre CM, Licht C, Muus P, et al. Terminal complement inhibitor eculizumab in atypical hemolytic-uremic syndrome. N Engl J Med 2013;368:2169-81. [Crossref] [PubMed]
- Licht C, Greenbaum LA, Muus P, et al. Efficacy and safety of eculizumab in atypical hemolytic uremic syndrome from 2-year extensions of phase 2 studies. Kidney Int 2015;87:1061-73. [Crossref] [PubMed]
- Holers VM. Contributions of animal models to mechanistic understandings of antibody-dependent disease and roles of the amplification loop. Immunol Rev 2023;313:181-93. [Crossref] [PubMed]
Cite this article as: Wu X, Liszewski MK, Java A, Atkinson JP. Atypical hemolytic uremic syndrome: genetically-based insights into pathogenesis through an analysis of the complement regulator CD46. Ann Blood 2023;8:27.